Drinking-Water Treatment Residuals to Control Phosphorus in Soils
Introduction
The use of drinking-water treatment residuals (WTR) to control excess phosphorus (P) in soils with limited P ad- sorption capacity has recently received increased attention. The target audience for this publication includes state agencies, like the Florida Department of Environmental Protection (FLDEP) and water management districts trying to control P pollution, and those interested in nutrient management for agricultural and environmental purposes.
Phosphorus Problem in Florida
Managed soils in Florida may contain excess soil phosphorus (P) concentrations as a result of repeated fertilizer, manure, or biosolids applications. Accumulation of P in soil does not damage soil fertility or harm plants but can promote off-site losses of P to surface water bodies. Water pollution due to excessive P is a major concern receiving much attention. Phosphorus moves from agricultural and urban areas either dissolved in water that drains away or as particulate matter (attached to soil particles) that travels with eroding soil. Increased P in water bodies is recognized as one of the major factors responsible for eutrophication-related decrease in water quality. Eutrophication is a term used to describe the process whereby a water body becomes rich in nutrients, particularly phosphate and nitrate, which promote the growth of algae. The decomposition of dead algae depletes the water body of oxygen and impedes the survival of other species.
Worldwide, most soils have a moderate to high capacity to retain P, which reduces P movement with drainage water. The term that describes the process by which a soil retains P is "sorption." In contrast to other areas, Florida has abundant soils that retain P poorly. Many of these soils are also very poorly drained, that is, they flood during periods of heavy rainfall. Low P sorbing capacity, in conjunction with a high water table, makes Florida soils vulnerable to P losses. Off- site P transport can also readily occur by leaching, which is the downward movement of P through the soil to the water table. Leached P can then move to surface waters via lateral subsurface flow as the soil drains internally.
The potential for a soil to lose P can be decreased if its P sorption capacity is increased through application of certain soil amendments. A municipal byproduct proven to be an effective amendment in controlling off-site P transport from poorly P-sorbing soils is drinking-water treatment residual (WTR), a byproduct of drinking-water purification. WTR naturally has a very high P sorption capacity, so researchers have proposed using it to reduce P loading into surface or ground water. Several best management practices (BMPs) using WTR to reduce nonpoint source pollution by P have been proposed. One approach is to surface-apply WTR to reduce transport of P in runoff water. Another approach is to incorporate WTR into soil to reduce the solubility of P accumulated from previous applications, preventing P leaching.
What are WTRs and how are they generated?
Drinking-water treatment residuals are primarily sediment, metal (aluminum, iron, or calcium) oxide/hydroxides, activated carbon, and lime removed from water during purification. Polymers are sometimes added to aid the dewatering process. The relative effectiveness of WTRs in reducing soluble P depends on several factors, including source water characteristics, water treatment methods and length of residual storage time prior to land application. Each water treatment facility uses unique source water and different treatment chemicals and processes, producing WTR with different physical and chemical compositions and P sorption capability (Table 1).
Coagulation, flocculation, and sedimentation (or coagulation and filtration) are processes used in water treatment to remove turbidity, color, taste, and odor from untreated water and to speed particulate matter removal. The purpose of coagulation and flocculation is to promote small particle combination and formation of larger aggregates. Typically, metal salts such as ferric chloride (FeCl3) or alum [Al2(SO4)3•14H2O] are used during the coagulation process to accomplish these steps. The residuals resulting from the use of iron or aluminum salts as coagulants are herein referred to as aluminum-based WTR (Al-WTR) and iron-based WTR (Fe-WTR). The other major residual type, calcium-based WTR (Ca-WTR), is produced in water-softening facilities where lime is used to decrease hardness.
How are WTRs disposed?
A consequence of the global expansion and proliferation of water treatment facilities is the increased generation of WTRs that require appropriate methods of disposal. There are thousands of drinking-water treatment plants in the United States that use metal salts as coagulants for efficient removal of particulate solids and colloids from surface water, generating more than 2 million metric tons of WTR daily. Drinking-water treatment residuals can be disposed a) directly to a receiving stream; b) to sanitary sewers; c) to a landfill, assuming that the residual contains no free-draining water and does not have toxic characteristics as defined by the toxicity characteristic leaching procedure (TCLP) test; and d) by land application. A 1991 national survey of 612 utilities serving populations of >50,000 showed that landfilling was the predominant disposal method, followed by land application, sanitary sewer disposal, direct stream discharge, and lagooning (Kawczyinski and Achtermann 1991). In Florida, the most acceptable long-term disposal methods for WTRs are landfilling and land application (O'Connor et al. 2002). The disposal (via landfill) cost of non-hazardous materials, including WTRs, is ~ $50 per metric ton, which can substantially increase the cost of treated drinking water. Furthermore, landfill space for WTR disposal will become increasingly limited in the future. Land application of WTR is therefore an attractive and relatively inexpensive alternative means of WTR disposal and may have the added benefit of immobilizing P in poor-sorbing soils.
Using WTR to Control Off-Site Phosphorus Losses
Land application of WTR has been shown to be cost-effective for effectively sorbing excess levels of labile P in soils. Laboratory studies have shown that Al-WTRs adsorb large amounts of P and increase the P-sorbing capacity of poorly P-sorbing soils, thereby decreasing P losses in runoff and leaching. For example, O'Flynn et al. (2012) demonstrated that alum and ferric chloride, the primary constituents of Al and Fe WTRs, respectively, reduced water extractable P in batch studies by >98%.
Surface application of WTR has been demonstrated to successfully reduce dissolved P concentrations in runoff water as well as in leachate. In a rainfall simulation study, Agyin-Birikorang et al. (2007a) reported a >50% reduction in P lost as runoff as well as via leaching relative to control soil without WTR amendment. These results are consistent with those reported by Silveira et al. (2013), which, in a 2-year field study, investigated groundwater P levels in field plots amended with Al-WTR compared to a control. Al-WTR decreased P leaching by >50%, whether surface-applied or incorporated, at 35 Mg ha-1 and by >80% at 70 Mg ha-1.
Agyin-Birikorang et al. (2008) also evaluated WTR effectiveness in a typical Florida spodosol (Immokalee fine sand) amended with different P sources (manure, biosolids and inorganic fertilizer) in a field study and observed decreases in soluble P concentrations of the P-source amended soil. Treatments without WTR amendments had greater water-extractable P (WEP) concentrations (>10 mg/kg) than the WTR amended treatments (Figure 1). In the absence of WTR, there were differences in WEP concentrations between different P-source treatments. However, with WTR amendment, not only were the WEP values significantly decreased, but the differences in the WEP concentrations arising from the differences among the P sources were no longer obvious (Figure 1).
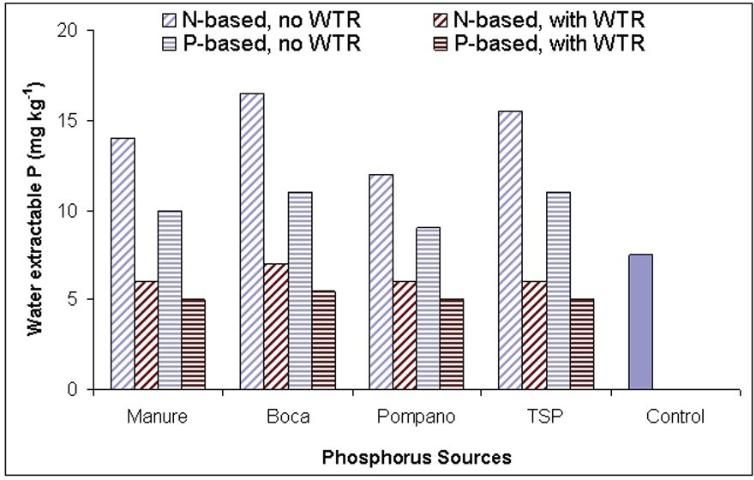
Beneficial use of WTR has also been expanded to reduce the solubility of P in organic soil amendments like manure or biosolids. For example, Silveira et al. (2006) investigated the use of Al-WTR to reduce P losses in a manure-impacted spodosol and reported that P leaching was reduced by up to 99.8% as a result of treatment with the Al-WTR.
Co-blending WTR with manure or biosolids before land application also reduces P solubility, as demonstrated by O'Rourke et al. (2012), who reported a >70% reduction in water extractable P after mixing WTR to manure at a rate of 144 g kg-1. Reducing P leaching in biosolids via amendment with WTR allows farmers to take advantage of the nitrogen, micronutrients, and organic carbon content of the manure or biosolids while reducing P loss risk.
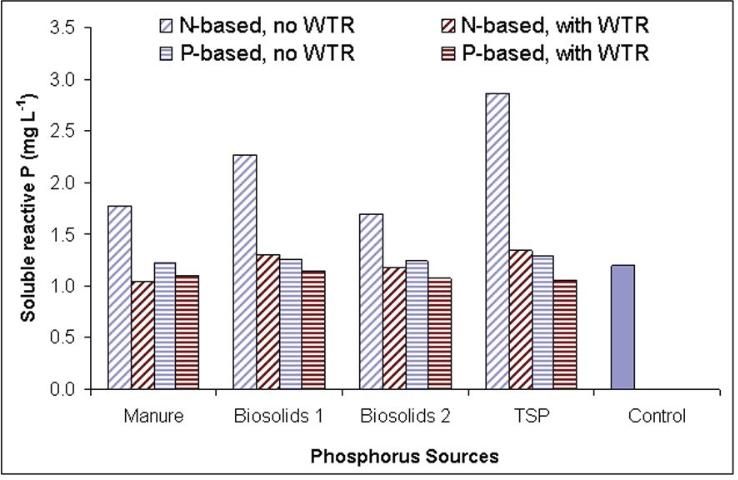
Can aluminum-based WTR retain P for a long time?
Concerns have been raised about the long-term stability of P that has been sorbed by a WTR soil amendment. We used methods such as spectroscopy and solid-state characterization of P-loaded WTR particles to better understand the long-term stability of sorbed P. The results suggest that P sorption by Al-WTRs is practically irreversible. P sorption to WTR is very strong, with little or no desorption observed. Thus, once immobilized by the WTR particles, P is likely irreversibly bound, barring destruction of the WTR particles associated with extremely low soil pH values.
Agyin-Birikorang and O'Connor (2007b) evaluated aging and pH effects on the lability of WTR-immobilized P using artificially aged WTR-amended Immokalee soil and field-aged Granby loamy sand. Within the pH range of commonly occurring agricultural soils (4 to 7), WTR amendment, coupled with aging, ultimately reduced labile P (as determined using method described in Morel and Torrent (1997)) in artificially aged samples by ~75%, and field-aged samples by about ~70% relative to the control (no-WTR) samples (Figure 3).
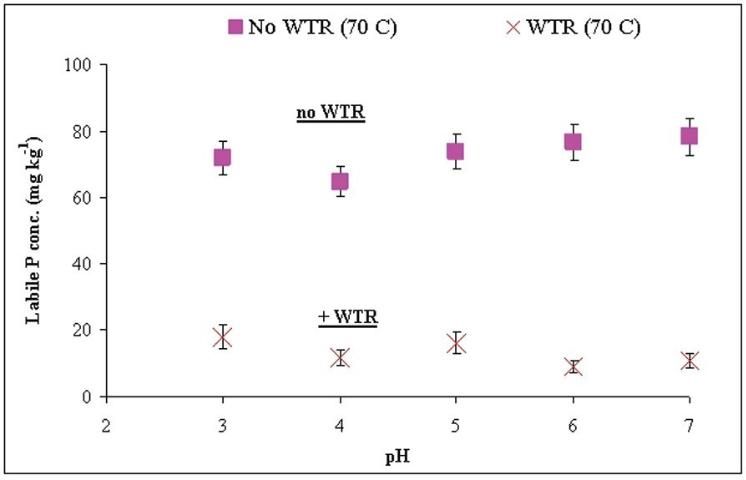
The results suggest that WTR application can reduce the labile P concentration in P-impacted soils for a long time within the pH range commonly encountered in agricultural soils. Determining the appropriate application rate of WTR is complicated due to variation in chemical properties influenced by the source of water, treatment chemicals and processing used by drinking-water treatment plants. Soils, and P-sources co-applied with WTR, can also vary in physical and chemical properties. Thus, the compositional variability of soils, P-sources (if co-applied with WTR), and WTRs need to be considered when determining WTR application rate. A quantitative approach using WTRs to reduce P flux from P-amended soils should be based on ensuring sufficient reactive Al + Fe in the WTR to immobilize labile P in the soil.
In a related field study in Michigan, Agyin-Birikorang et al. (2007) assessed the longevity of an Al-WTR immobilization of P in two fields with long histories of poultry manure applications. The authors utilized rainfall simulation techniques to investigate P losses in runoff and leachate from soils amended with a one-time application of an Al-based WTR 7.5 yr earlier. Amendment with Al-WTR reduced soluble P concentration in the soils by >60% compared with control plots, and the WTR-immobilized P remained stable for at least 7.5 years. Amendment with Al-based WTR reduced total dissolved P and biologically available P by >50%, showing that the WTR-immobilized P indeed remained non-labile (Agyin-Birikorang et al. 2007).
Factors to Consider When Applying WTR
Different WTRs can have different chemical characteristics (including different P sorption capacities), and application of different WTRs at the same rate can result in different agronomic and/or environmental impacts. Haustein et al. (2000) compared the potential of two Al-rich materials to reduce runoff P from excessively P-impacted fields. The material with greater total Al concentration (46.7 g/kg), applied at both 9 and 18 metric tons/hectare, decreased runoff P below that measured in control plots throughout the 4-month experiment. However, at the same WTR application rates, the material with a lower total Al concentration (15.9 g/kg) decreased the runoff P for only one month (Haustein et al., 2000).
Determining the appropriate application rate of WTR is complicated due to variation in chemical properties influenced by the source of water, treatment chemicals and processing used by drinking-water treatment plants. Soils and P-sources co-applied with WTR can also vary in physical and chemical properties. Thus, the compositional variability of soils, P-sources (if co-applied with WTR), and WTRs need to be considered when determining WTR application rate. A quantitative approach using WTRs to reduce P flux from P-amended soils should be based on ensuring sufficient reactive Al + Fe in the WTR to immobilize labile P in the soil.
Nair and Harris (2004) developed a technique by which to predict the amount of P a soil can sorb before exceeding a threshold soil equilibrium concentration, or soil phosphorus storage capacity (SPSC). SPSC values are calculated from oxalate-extractable P, Fe, and Al concentrations of a soil using the following equation:
SPSC (mg P/kg) = (0.15 - PSR) * (Alox + Feox) * 31

and Pox, Alox, and Feox are 0.2 M oxalate-extractable P, Al, and Fe concentrations of the soil respectively (expressed in mmoles). SPSC values range from negative values (for highly P-impacted soils with no remaining P retention
capacity) to positive values (for less P-impacted soils, excess P retention capacity). Oladeji et al. (2007) identified zero SPSC as an agronomic threshold above which yields and P concentrations of plants may decline and below which there is little or no yield response to increased plant P concentrations. The consensus among researchers is that soils can be managed to maintain soil test P for optimal economic crop yields while minimizing the risk of offsite P loss. Applying P sources at any rate, along with sufficient WTR to give a SPSC value of 0 mg/kg, enhances environmental benefits (reduced P loss potential) without negative agronomic impact.
An application of WTR based on the SPSC threshold targets only the excess P that poses an environmental threat and is not expected to negatively impact plant-available soil P needed to meet the plant requirement. Therefore, P storage capacity should be determined for WTRs and P sources (if any) prior to land application. The P storage capacity of the WTR and the P sources can be determined by modifying the SPSC equation (above) by substituting PSR with the phosphorus saturation index (PSI). (The PSI is equivalent to PSR but the term PSI is used to describe P binding/release potential of organic sources, whereas PSR is applied when describing soils). Thus, the P storage capacity of the P sources (APSCsource) and WTR (APSCWTR) can be calculated as:
APSCSource (mg P/kg) = [(0.15-PSI)*(Alox + Feox)]*31
APSCWTR (mg P/kg) = [(0.15-PSI)*(Alox + Feox)]*31

The P storage capacity of the soils and amendments can then be combined to determine the amount of WTR to apply to a P impacted soil or to be co-applied with other P sources using the following equation:

The SPSC value of the soil and the APSC values of P-source and the WTR can be determined from the chemical compositions of the soil and amendments. The quantity
of the P-sources is known from the application rate, and the mass of soil could be determined from the land area to depth of impact (depending on application method; 15 cm depth if incorporated, or 5 cm when surface applied) and the soil bulk density. The only unknown in the equation would be the mass of WTR, which can be determined by substituting the known values into the equation.
The SPSC-based WTR application rate will not only account for the P, Al, and Fe concentrations in the residuals and the soil, but the threshold soil P value as well. Thus, the WTR rate required to attain a desired SPSC value can be calculated to ensure a soil P concentration below the environmental threshold, while at the same time supplying sufficient P to meet plant needs.
Further Readings
Agyin-Birikorang, S., G. A. O'Connor, L. W. Jacobs, K. C. Makris, and S. R. Brinton. 2007. "Long-Term Phosphorus Immobilization by a Drinking Water Treatment Residual." J. Environ. Qual. 36:316–323.
Agyin-Birikorang, S., and G. A. O'Connor. 2007b. "Lability of Drinking-Water Treatment Residuals (WTR) Immobilized Phosphorus: Aging and pH Effects." J. Environ. Qual. 36:1076–1085.
Agyin-Birikorang, S., G. A. O'Connor, O. O. Oladeji, T. A. Obreza, and J. C. Capece. 2008. "Drinking-Water Treatment Residuals (WTR) Effects on the Phosphorus Status of Field Soils Amended with Biosolids, Manure, and Fertilizer." Commun. Soil Sci. Plant Anal. 39: 1700–1719.
Haustein, G. K., T. C. Daniel, D. M. Miller, P. A. Moore, Jr., and R. W. McNew. 2000. "Aluminum-Containing Residuals Influence High Phosphorus Soils and Runoff Water Quality." J. Environ. Qual. 29: 1954–1959.
Kawczyinski, E., and V. Achtermann. 1991. "A Water Industry Database Report on Residuals Handling." In Proc. of the AWWA/WEF Joint Residuals Conf. Durham, NC. 11-14 Aug. American Water Works Association, Denver, Colorado, p. 6b-1 to 6b-5.
Makris, K. C., W. G. Harris, G. A. O'Connor, and T. A. Obreza. 2005. "Long-Term Phosphorus Effects on Evolving Physicochemical Properties of Iron and Aluminum Hydroxides." J. Colloid Interface Sci. 287:552–560.
Morel, C., and J. Torrent. 1997. "Sensitivity of Isotopically Exchangeable Phosphate in Soil Suspensions to the Supporting Solution." Soil Sci. Soc. Am. J. 61: 1044–1052.
Nair, V. D., and W. G. Harris. 2004. "A Capacity Factor as an Alternative to Soil Test Phosphorus in Phosphorus Risk Assessment." New Zealand J. Agri. Res. 47:491–497.
O'Flynn, C. J., O. Fenton, and M. G. Healy. 2012. "Evaluation of Amendments to Control Phosphorus Losses in Runoff from Pig Slurry Applications to Land." Clean–Soil, Air, Water 40(2): 164–170.
Oladeji, O. O, G. A. O'Connor, J. B. Sartain, and V. D. Nair. 2007. "Controlled Application Rate of Water Treatment Residual for Agronomic and Environmental Benefits." J. Environ. Qual. 36:1715–1724.
O'Rourke, S. M., R. H. Foy, C. J. Watson, A. Gordon, and D. Doody. 2012. "Assessment of Co-Blending Water Treatment Residual with Dairy Manure to Reduce Phosphorus Concentrations in Runoff in Northern Ireland." Soil Use and Management 28(2): 157–166.
Silveira, M. L., M. K. Miyittah, and G. A. O'Connor. 2006. "Phosphorus Release from a Manure-Impacted Spodosol: Effects of a Water Treatment Residual." J. Environ. Qual. 35(2): 529–541.
Silveira, M. L., J. L. Driscoll, C. P. Silveira, D. A. Graetz, L. E. Sollenberger, and J. Vendramini. 2013. "Land Application of Aluminum Water Treatment Residual to Bahiagrass Pastures: Soil and Forage Responses." Agronomy Journal 105(3): 796–802.