Hydrologic Control of the South Florida Landscape
Water-control efforts during the 20th century created major changes in the south Florida landscape, dramatically altering hydrology and ecology while enabling rapid agricultural and urban development (Figure 1). Historically, Lake Okeechobee, the sawgrass plains, ridge and slough habitats of the Everglades, the cypress swamps and prairies of the Big Cypress, and the mangrove swamps along the coasts were connected hydrologically and were shaped by the steady south/southwestward flow of a wide sheet of shallow water (Light and Dineen 1994; SCT 2003). Today, water depth, flow, and flooding duration in the region result from a combination of seasonal rainfall and a management regime that attempts to balance ecological needs with those of agriculture and the growing population of south Florida's ever-expanding urban areas.
Canals and levees are the foundation of the south Florida water-management infrastructure. Although small-scale, shallow canals existed in the Everglades as early as A.D. 300—built by the native Ortona and, later, Calusa and Tequesta people to connect villages to coastal trade routes (Carr et al. 2002; MacMahon and Marquardt 2004; NPS 2010; Figure 2)—modern canals are wider, deeper, and hundreds of kilometers longer than the pre-Colombian navigation trails (Light and Dineen 1994, Carr et al. 2002).
Four major drainage canals (West Palm Beach, Hillsboro, North New River, and Miami), totaling 380 km (236 miles), were dredged through the Everglades in the early 20th century (Figure 3), starting an era of hydrological modification. Material from canal dredging was used to erect levees for the purpose of impounding waters and to raise roadbeds to avoid flooding. Drainage efforts, combined with the construction of the Tamiami Trail canal and levee (1915–1928), substantially altered the hydrology of the region: water levels were lowered throughout the Everglades basin, and the natural north-to-south flow pattern was interrupted (Light and Dineen 1994, United States Army Corps of Engineers [USACE] and South Florida Water Management District [SFWMD] 2002). Major hurricanes in 1926 and 1928, and again in 1947–48, claimed thousands of lives and underscored the need for a comprehensive water-management system.
The Central and Southern Florida Project for Flood Control and Other Purposes (C&SF Project) was authorized by the US Congress in 1948 to provide flood control and water supply to urban and agricultural areas, and to address the problems of saltwater intrusion and uncontrollable muck fires caused by drainage (Light and Dineen 1994; Lodge 2010). The C&SF Project constructed levees, pumps, and water storage areas, and expanded and deepened the canal system. The project provided many of its intended benefits; however, it exacerbated the Everglades' ecological problems by further disrupting sheet flow and diverting vast quantities of fresh water away from wetlands and southern estuaries, and redirecting it to the northern estuaries through artificial connections. The Comprehensive Everglades Restoration Plan (CERP) was authorized in 2000 to undo some of the ecological damage by attempting to restore a more natural water flow through the Everglades, while enhancing water supplies for south Florida's cities and farmers.
As a result of these management changes in south Florida's environment, canals and levees have become part of the region's hydrologic, ecological, and recreational landscape. For instance, south Florida's canals are listed as among the state's best freshwater recreational fisheries (FWC 2010a). In a 2008–2009 survey, the L-67A Canal (Water Conservation Area 3) had the highest catch rate for both largemouth bass and sunfishes and accounted for 80% of the total largemouth bass fishing effort across 17 water bodies surveyed in the state (Johnson 2009). Everglades canals also provide access for hunters, and the levees are used for hiking, biking, and camping (FWC 2010b).
Canals and levees can act to either increase or decrease connectivity at the landscape scale. As barriers, they limit and redirect surface water flow and limit the movements of species, energy, and ecosystem processes such as fire. As conduits, they can enhance interactions between surface water and groundwater and facilitate the persistence and rapid spread of nutrients, pollutants, and nonnative species. In addition, canals serve as habitat suitable for animals and plants that require deep water, either year-round or seasonally. Despite the growing body of research on Everglades ecosystem structure and function over the past 20 years, no data to date support the notion that fragmentation and compartmentalization resulting from canals is beneficial for the system. On the contrary, many of the factors demonstrated to contribute to the degradation of the ecosystem are tied directly to the structural components of the C&SF Project and their effects of drainage and impoundment (Davis and Ogden 1994, McIvor et al. 1994, Sklar et al. 2002, SCT 2003).
Though the effects of canals and levees are felt throughout the Greater Everglades ecosystem in urban, agricultural, and natural areas, the focus of this document is on the natural areas of the Everglades Protection Area (EPA), Big Cypress Swamp, and coastal wetlands. The EPA includes the three Water Conservation Areas (WCAs), including the Arthur R. Marshall Loxahatchee National Wildlife Refuge (LNWR), and Everglades National Park (ENP) which, combined, represent the largest remaining area of natural Everglades. CERP targets restoration of these areas. Decompartmentalization (restoring the historic "river of grass" by removing or modifying levees, canals, and other barriers to sheet flow) is a central priority of CERP (USACE and SFWMD 2002). The purpose of this paper is to comprehensively portray the hydrological and ecological effects of canals and levees within the EPA landscape and to consider ways their impacts may be mitigated.
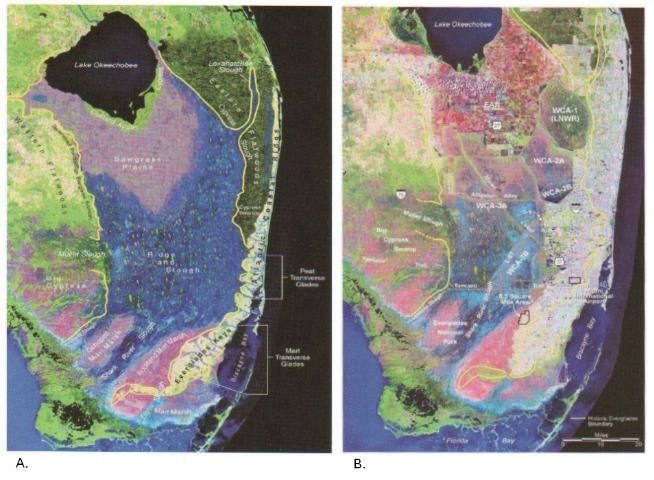
Credit: SFWMD via Lodge (2010)
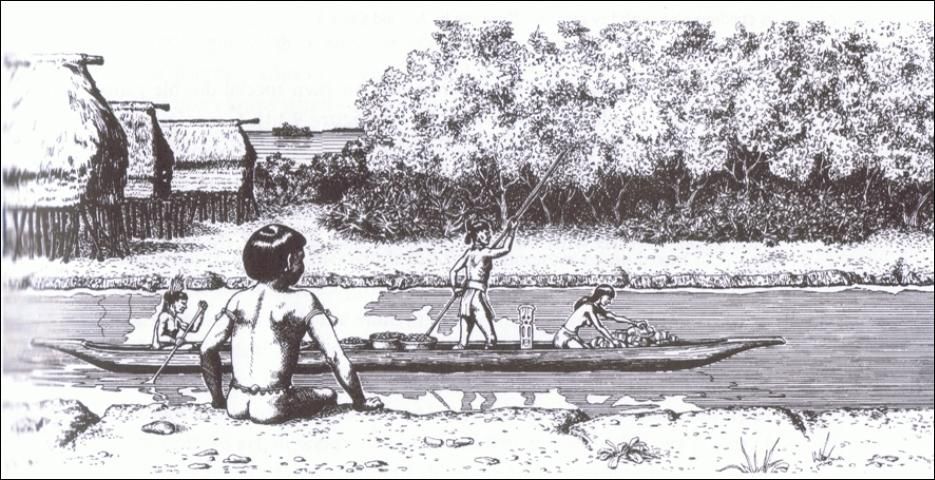
Credit: Merald Clark. Source: MacMahon and Marquardt (2004)
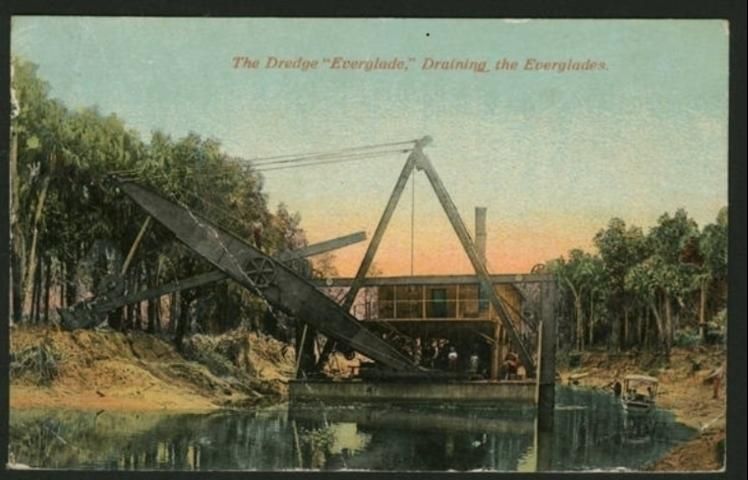
Credit: Light and Dineen 1994
Effects of Canals and Levees on Hydrology
Canals and levees in the Everglades were typically constructed together as levee-canal complexes because of the need for borrow pits when constructing levees and disposal sites when constructing canals. Levees were built for the primary purpose of storing water during dry periods and restricting seepage into developed but low-lying areas located between the Everglades and the densely populated Atlantic coastal ridge. Canals were constructed to drain and reclaim the wetlands and to convey water to southeastern Florida where it recharges into the aquifer to supply well fields for the urban population. During times when water is plentiful and the threat of flooding exists, the same canals are used to discharge fresh water from the Everglades to the coasts.
As intended, canals built in the early 20th century and later expanded by the C&SF Project have diverted enormous amounts of water from south Florida's natural areas for urban use and flood control. Approximately 6.4 billion liters (1.7 billion gallons) of water are discharged daily to the Atlantic Ocean and the Gulf of Mexico (CERP 2010). According to South Florida Water Management District (SFWMD) models, the four major drainage canals remove more than twice the amount of net annual precipitation that falls in the Everglades (Sklar et al. 2002). Since canal construction began, more than 50% of south Florida's wetlands have been lost and water tables in much of the remaining Everglades have been lowered (Figure 4), resulting in the loss of peat soils, loss of coastal well fields from saltwater intrusion, and salinization of formerly oligohaline (low salinity) wetlands (e.g., Cape Sable; D.C. Tabb, University of Miami, pers. comm.; Loftus and Kushlan 1987; Davis and Ogden 1994; Sonenshein 1996; Wanless and Vlaswinkel 2005). Depletion of natural water storage areas and flow losses by canal discharge to the coast are thought to be major factors underlying the persistence of overly dry conditions in the Everglades south of Tamiami Trail.
The diversion of water from Shark River Slough and Taylor Slough in the southern Everglades has significantly reduced freshwater inflow to Florida Bay and led to hypersalinity in biologically vital coastal estuaries (Fourqurean et al. 1993; McIvor et al. 1994; Nuttle et al. 2000). In addition, fresh water is no longer delivered by gradual sheet flow but via canals in sudden, unnaturally timed pulses (Light and Dineen 1994; Abtew et al. 2010). These sudden releases flood wildlife habitat, disperse fish concentrations, and dramatically alter salinities in estuaries, resulting in mortality of estuarine species (McIvor et al. 1994; Sklar and Browder 1998; Lorenz 2000). For instance, pulsed discharges and rapid salinity fluctuations in coastal Biscayne Bay caused important changes in fish assemblages, decreasing numbers and species richness (Serafy et al. 1997).
Levees and canals have other less obvious but potentially damaging hydrologic effects on Everglades ecosystems. Levees create deep, pooled conditions in southern areas of the enclosed basins, with minimal or no flow (Figure 5). The average water depths tend to be too deep to support a diverse assemblage of plant communities (Watts et al. 2010). Also, the excavation of canals through the less permeable peat into the more highly permeable aquifer, and the "stair-step" of abrupt water-level changes created by levees, enhance groundwater-surface water interactions in the Everglades (Krupa and Diaz 2002; Harvey et al. 2004). Both levee and canal operations increase upward mixing and discharge of relatively salty water from deep in the sand and limestone aquifer beneath the Everglades, which is damaging to sensitive biological communities (Harvey and McCormick 2009).
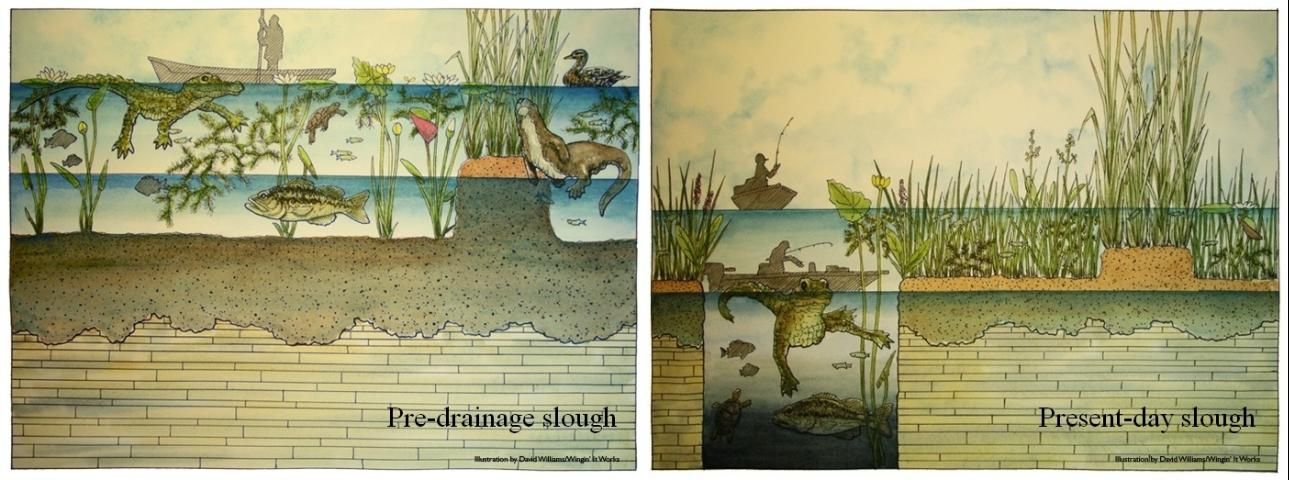
Credit: Christopher McVoy, SFWMD
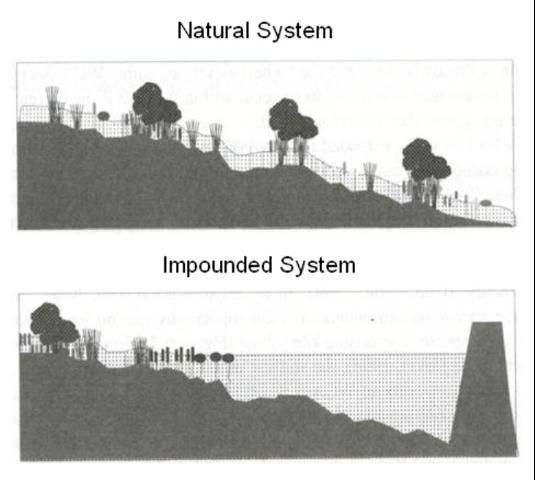
Effects of Canals and Levees on Water Quality
Nutrient Enrichment
A major impact on Everglades structure and function has been the delivery of agricultural nutrients, especially phosphorus, into wetlands by the canal system. Small quantities of that fertilizer component have caused dramatic changes in algal/vegetation composition and structure of these wetlands, which evolved under very nutrient-poor conditions (Noe et al. 2001). Everglades canals have been found to contain concentrations of phosphorus up to 30 times those historically present in the marsh (McCormick et al. 1996). Phosphorus concentrations are highest in the northern Everglades and decrease along a north-to-south gradient that extends up to 7 km into the surrounding marsh (McCormick et al. 1996). Thus, enrichment and linked ecological processes vary through the marsh as a function of distance to canals (Doren et al. 1997; Childers et al. 2003; Rehage and Trexler 2006). The most pronounced vegetation change resulting from phosphorus enrichment occurred in WCA 2A, which receives water directly from the Everglades Agricultural Area (EAA) (McCormick et al. 2002; Rutchey et al. 2008). In LNWR the nutrient-enriched discharges from EAA tend to be confined to the marsh perimeter, while canal inflows into the other WCAs and ENP have considerably lower phosphorus concentrations than those in the northern Everglades. However, in ENP they are still elevated compared with reference sites in the interior of the park (McCormick et al. 2002; NPS 2005).
Additions of phosphorus have led to the replacement of periphyton/Utricularia mats with filamentous algal species that thrive in enriched waters, and the shift from a sawgrass-dominated vegetation community to one dominated by cattails (Typha domingensis) (McCormick et al. 2002; Sklar et al. 2002). Cattails limit light penetration, further reducing periphyton growth (Grimshaw et al. 1997), decreasing oxygen availability (McCormick and Laing 2003), and resulting in changes in invertebrate and fish community structure (Turner et al. 1999, Noe et al. 2001; McCormick et al. 2002; McCormick et al. 2004).
Chemical Contaminants
Canals have been implicated in the introduction of excess chemicals from urban and agricultural areas into the Everglades (Gunderson and Loftus 1993). The SFWMD's ambient pesticide monitoring program, which has routinely tracked surface water and sediment in canals since 1984, has found pesticides at detectable levels at almost every monitoring site, with herbicide compounds, particularly ametryn and atrazine, found most frequently in surface water and DDE and DDD (metabolites of the banned pesticide DDT) detected most frequently in sediments (Pfeuffer and Rand 2004). Levels of some insecticides, including endosulfan which is very toxic to aquatic fauna, at times exceed state surface water quality standards (Pfeuffer and Rand 2004). Carriger et al. (2006) identified five pesticides of potential ecological concern in south Florida canals (based on their exceedence of state sediment quality standards): DDT, DDD, DDE, chlordane and endosulfan. More recently, a risk assessment of nine herbicides found throughout south Florida's freshwater ecosystems, reports that risk associated with individual chemicals was low, but risk associated with herbicide mixtures (mainly bromacil, diuron, and norflurazon) was high in certain areas (Schuler and Rand 2008). Pesticide residues have also been detected outside of canals in the surface waters of Florida Bay, with evidence of toxicity to estuarine organisms (Scott et al. 2002).
In the 1990s, mercury was found at dangerous levels in Everglades fishes and their predators (Stober et al. 1995). Bates et al. (2002) implicated high levels of sulfur delivered into wetlands by canal discharge as a mediator in methylation of mercury. Methylated mercury is at least 10 times as toxic and bioaccumulative as is elemental mercury. While accumulation of methylmercury occurs mostly in the marsh (rather than in canals), canal fish become contaminated when they consume marsh prey forced into canals by low water or when they forage in the marsh during the wet season. Mercury levels in fish and birds have declined markedly since the 1990s (Frederick et al. 2005), but methylmercury is still at levels high enough to affect reproduction by wading birds in many parts of the Everglades (Frederick and Jayasena in press).
Changes in Conductivity
Degradation of Everglades water quality is indicated by increases in the water's conductivity (i.e., its ability to carry an electrical current), which is affected by presence of inorganic dissolved solids such as chloride, sulfate, sodium, and calcium. Canals dug into the surficial aquifer, and levees that created a "stair-step" of water levels, have increased the relative contributions of both groundwater and surface-water inflows (both of which are higher in ionic strength than precipitation) to Everglades hydrologic budgets (Harvey and McCormick 2009). From 1959 to 1979, as inflow into Shark River Slough changed from being dominated by unregulated marsh flow to canal discharge, wet season specific conductance rose from 250 to 600 µmhos/cm (Flora and Rosendahl 1982). Currently, water in the Everglades Agricultural Area drainage canals can exceed 1,000 µmhos/cm and pronounced conductivity gradients occur throughout the Everglades canals into the marsh (Scheidt et al. 2000). Specific conductivity within the LNWR interior marsh is often less than 100 µmhos/cm, even during the dry season, whereas in WCA 2A it often exceeds 800 µmhos/cm as a result of discharges of stormwater and groundwater from the Everglades Agricultural Area (Scheidt et al. 2000; Harvey and McCormick 2009).
Specific conductivity in WCA 2A often exceeds 800 µmhos/cm as a result of stormwater and groundwater discharges from the Everglades Agricultural Area, whereas in the LNWR interior marsh it is often less than 100 µmhos/cm, even during the dry season (Scheidt et al. 2000; Harvey and McCormick 2009). However, in LNWR, when stages in the perimeter canal rise above interior marsh stages as a result of stormwater discharges, canal water can intrude as much as 3.9 km into the unimpacted Refuge interior (Surratt et al. 2008). LNWR's expanded water quality monitoring program uses the higher conductivity of the canal water as a conservative tracer of canal water movement into the soft-water marsh.
Effects of Canals and Levees on Landscape Features and Biota
Wetland Fragmentation
Degradation of Ridge and Slough Landscape
Canals and levees fragment what were once continuous wetlands and interrupt historical sheet flow across the landscape (Sklar et al. 2002; SCT 2003; Ogden 2005; Larsen and Harvey 2010). The existing system does not contain a functional landscape mosaic of adequate extent, heterogeneity, spatial configuration, connectivity, and natural hydrologic periodicity to provide the seasonal habitat requirements of historical populations of alligators, wading birds, snail kites, and their prey. Connectivity of contiguous marsh areas is particularly important during and following dry-down events, so that dispersal and recolonization of affected marsh areas by aquatic organisms can occur and aquatic productivity may be maintained (Fleming et al. 1994).
Compartmentalization of the Everglades has led to soil loss, flattening of the peat surface, and altered flow velocity, resulting in degradation of the distinct directional pattern of ridge and slough vegetation (Sklar et al. 2002, Larsen et al. 2007). Much of the post-drainage landscape is scattered, blurred, and unstructured with major changes in plant communities (SCT 2003; Figure 6). The mosaic landscape has been replaced in many areas by large uniform stands of sawgrass (SCT 2003; Figure 6), which are much poorer in aquatic species than the sloughs and wet prairies that preceded them (Loftus and Kushlan 1987; Gunderson and Loftus 1993). Thus, the compartmentalized landscape offers considerably less habitat, foraging areas, and refuges for wildlife. Wading birds, often considered indicators of the health of Everglades ecosystems, thrive in a mosaic of wetland habitats that includes wet prairies and sloughs, since they rarely use dense sawgrass stands (Hoffman et al. 1994). The endangered Everglades snail kite (Rostrhamus sociabilis plumbeus) also depends on a diversity of wetland habitats. Relying almost exclusively on a single prey species (the apple snail, Pomacea paludosa), snail kites forage mainly in sparse emergent vegetation in open-water areas (Bennetts et al. 1994). Thus the vanishing of the ridge and slough matrix may further threaten this endangered species.
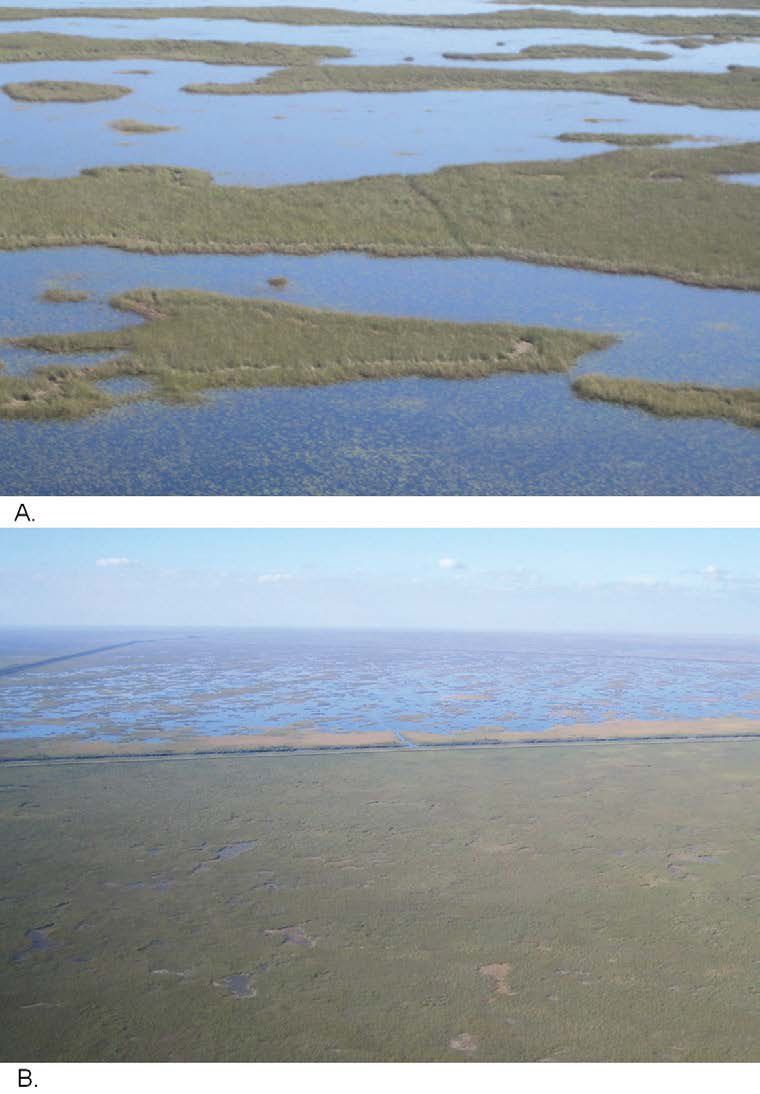
Credit: Christopher McVoy, SFWMD
Degradation of Tree Islands
Loss of flow also appears to have a negative impact on tree islands scattered throughout the ridge and slough matrix. Tree islands are biodiversity hotspots that provide food, cover, and critical nesting sites for numerous species; they also play an important ecological role in the sequestration of carbon and phosphorus (Sklar and Van der Valk 2002). Since compartmentalization, tree island area has declined by 61% in WCA 3 (1940–1995; Patterson and Finck 1999), and by 87% in WCA 2 (1953–1995; Hofmockel 1999). The once tear-shaped islands (oriented approximately north-south according to water flow,) are becoming irregularly shaped (Brandt et al. 2000), and are losing peat soil depth and elevation (Sklar and van der Valk 2002).
Barriers to Gene Flow
Fish and aquatic invertebrate community structure varies considerably across WCAs 3A and 3B, Northeast Shark Slough, and the rest of ENP, which may not be necessarily expected in the absence of canals and levees (Trexler et al. 2002; Chick et al. 2004; Trexler et al. 2005; Rehage and Trexler 2006). Compartmentalization of the system may also appear to affect the genetic structure of certain aquatic taxa populations, despite the fact that only about 60 generations have passed since canal and levee construction (Trexler and Loftus 2005). However, populations of at least one species, the spotted sunfish (Lepomis punctatus), did not show genetic differentiation, possibly as a result of sub-populations mixing in the canal system during the dry season (McElroy et al. 2003).
Barriers to Disturbance
Canals and levees also function as barriers to disturbance. Lightning-ignited wildfires were a certain and predictable landscape process in pre-20th century Florida (Abrahamson and Hartnett 1990). While the incidence of lightning may not have changed, the probability that lightning-ignited fire can propagate across a large area of the remnant Everglades landscape has decreased because of the increased number of firebreaks from canals, levees, and roads (Abrahamson and Hartnett 1990; Sklar et al. 2002).
Spread of Nonnative Species
Nonnative Fishes
Since the 1950s, when pike killifish (Belonesox belizanus) and oscars (Astronotus ocellatus) were first recorded in south Florida canals (Belshe 1961), more than 50 exotic fish species have been introduced into the region's fresh waters (Courtenay 1997, Fuller et al. 1999). In the most recent assessment, 34 nonnative freshwater fish species were found to be reproducing in Florida, 23 of which were considered established (Shafland et al. 2008). Canals facilitate establishment of these species by offering permanent thermal and drought refugia habitats (Trexler et al. 2000; Schofield et al. 2010). During cold snaps, nonnative tropical fishes may encounter lethal temperatures (between 6 and 15°C, depending on the species) in the marsh but not in the deep canals (Shafland and Pestrak 1982, Loftus and Kushlan 1987, Shafland 1995, Schofield et al. 2010). Figure 7 displays water temperatures in ENP marsh and alligator-hole habitats, compared to a nearby canal (L-31W), during the record cold event of January 2010; temperatures fell below 15°C in the marsh and alligator-hole habitats, but not in the canal.
A summary of eight quantitative fish surveys in southern Florida (Trexler et al. 2000) concluded that introduced species were most abundant in canals (particularly canals in urban southeastern Florida and those lacking connections to wetlands), in estuarine areas of the southern Everglades (where winter temperatures are mildest), and in solution holes in the Rocky Glades (dry-season refugia where nonnatives often outnumber native fish) (Kobza et al. 2004; Loftus et al. 2006). Recent monitoring shows that up to 70% of the fish community of canals can be composed of nonnative fishes (Gandy and Rehage unpubl. data), but significant variability was detected among canals, suggesting that either dispersal opportunities or habitat quality varies strongly among them. The relatively lower abundance of non-indigenous fishes in wet prairies and marshes distant from canals suggests that some species may not be well suited to native freshwater Everglades habitats and/or their varying hydrological regime (Trexler et al. 2000). However, the number of invasions continues to increase and, at least in ENP, appears associated with new water-management practices intended to restore hydrologic conditions and increase the hydroperiod of marl marshes (Kline et al. 2008).
To date, few studies have documented significant detrimental ecological effects from these introductions, leading to conflicting perspectives on the overall impact of nonnative aquatic taxa in the Everglades ecosystem (Shafland 1996; Trexler et al. 2001). Yet, as the number of invasions continues to increase (e.g., 5 new nonnative fish species in ENP since 2000; Kline et al. 2008), the potential increases for significant impacts that may alter ecosystem structure and function, and the ability of the system to provide for key ecosystem services (i.e., recreational fisheries). Evidence is increasing but still inconclusive for some species as to whether introduced fishes harm native aquatic communities in south Florida (Shafland 1996; Trexler et al. 2000; Baber and Babbit 2003; Loftus et al. 2006; Brooks and Jordan 2009; Rehage et al. 2009). Any lack of harm may be because native aquatic fauna tend to be habitat generalists that are resilient to both natural and anthropogenic disturbances (Trexler et al. 2000). On the other hand, there may be impacts on native species that are as yet unseen (Courtenay 1997), beyond the detection ability of current monitoring programs (Trexler et al. 2000), or unknown due to the fact that we lack pre-invasion data. At the same time, there is no doubt that the presence of nonnative fishes has altered ecosystem structure. For instance, fish diversity in ENP has increased by 40% with the addition of 14 established nonnative fishes (Shafland et al. 2008). The question remains, however, whether these changes in structure result in detectable and significant changes in ecosystem function and the provisioning of services. More research, particularly empirical work, is needed to tackle this question.
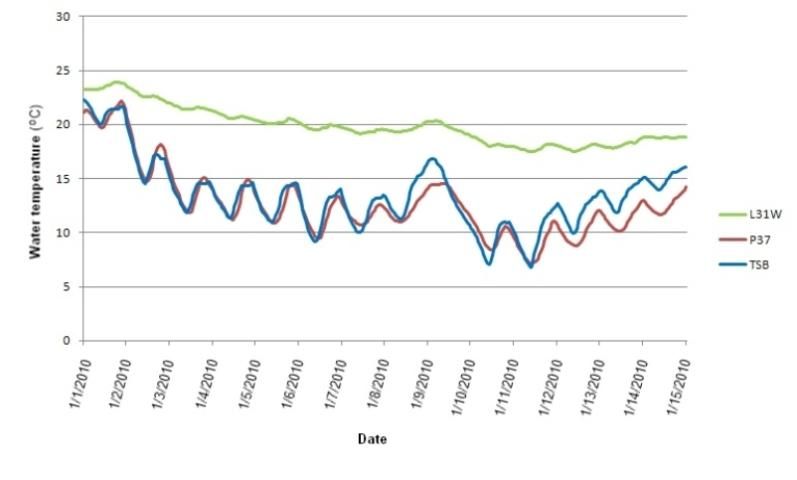
Credit: SFNRC (2010)
Nonnative Aquatic Invertebrates
Canals also appear to be the pathway for dispersal of potentially harmful nonnative invertebrates (five species of snails and one clam). The exotic red-rimmed melania snail (Melanoides tuberculatus) has been established in ENP since the 1970s, and was recently discovered in Biscayne National Park (BNP) in 2003. All specimens found in BNP have been concentrated near canal mouths and in nearshore areas between canals, suggesting that the snails are dispersing through canals (Wingard et al. 2008). The red-rimmed melania carries parasitic liver flukes and lung flukes that can cause illness in animals and humans who consume infected fish or crabs. In addition to potential health and economic effects, this species may cause major ecological damage by out-competing native snail species (Wingard et al. 2008). Exotic apple snails (Pomacea insularum, P. halstrum, and P. diffusa) and the giant ramshorn snail (Marisa cornuarietis) continue to spread throughout the Everglades, and particularly in the vicinity of canals. The island apple snail, P. insularum, competes with the native Florida apple snail (P. paludosa) (Rawlings et al. 2007) and, because of its larger size, may reduce the foraging success of the endangered Everglades snail kite (Darby et al. 2007; Cattau et al. 2010). Nonnative apple snails are also carriers of the rat lungworm, a parasite that infects humans exposed to the snails (Hollingsworth and Cowie 2006).
Nonnative Aquatic Plants
Canals enhance growing conditions for establishment and expansion of three major invasive pest plants: water lettuce (Pistia stratiotes), hydrilla (Hydrilla verticillata), and water hyacinth (Eichhornia crassipes), as well as several other widespread species in the aquarium trade, such as Hygrophila spp. and Rotala rotundifolia. Canals allow for the distribution and expansion of pest plants from urban areas to the east, and provide ample deep-water, nutrient-enriched habitats for them. Water lettuce, water hyacinth, and hydrilla have been shown to modify water chemistry, slow the already limited water flow in canals, and shade out native species (Schmitz et al. 1993). Water hyacinth also increases detritus-deposition rates, which depletes oxygen levels and can lead to large-scale mortality of aquatic organisms (Schmitz et al. 1993). In addition, the dense vegetation created by these aquatic invaders can impede navigation, flood control, and the recreational use of canals (Cervone et al. 2004). Each year, millions of dollars are spent for invasive plant management in Florida's canal systems. In 2002, the SFWMD spent $2 million to manage more than 9,712 ha (24,000 acres) of nuisance plants in canals (Cervone et al. 2004).
Terrestrial Invaders
Levees associated with canals provide disturbed upland habitat for the most noxious terrestrial pest plants in south Florida, most notably Australian pine (Casuarina equisetifolia), Brazilian peppertree (Schinus terebinthifolius), and several exotic grasses such as Burma reed (Neyraudia reynaudiana) and napier grass (Pennisetum purpureum). Recent vegetation mapping by the University of Georgia demonstrates that levees are corridors for nonnative plant persistence and dispersal (Forman and Alexander 1998). The persistence of pest plants on levees makes them available for dispersal into pristine habitat by "island hopping." In addition, levees are barriers to the dispersal of native water-dispersing marsh plants and aquatic animals. Levees also act as artificial terrestrial corridors into the wetland landscape for insect species such as fire ants (Ferriter et al. 2004), and recent surveys show that levees in the wetlands offer basking and nesting sites for invasive Burmese pythons (R.W. Snow, ENP, pers. comm.).
Habitats, Refuges, and Sinks
The habitat value of canals is a complex topic that is receiving increasing attention of researchers (J.L. Kline, ENP, pers. comm.; J.S. Rehage and J.C. Trexler, Florida International University, pers. comm.). Despite the fact that canals are a long-standing feature of the Everglades, our understanding of how they function as habitat for aquatic fauna and how this may be similar or different from natural habitats is still very limited.
Pathway into Interior Wetlands
Without canals, many new colonists and marine invaders would not successfully colonize interior wetlands. These deep-water habitats connect freshwater areas to the coast, allowing fishes tolerant of fresh and brackish waters to move far inland. Through connections to the northern Everglades, canals have also received colonists from that region, thereby boosting the numbers of native species in canals and allowing for range expansion among native fishes (Loftus et al. 2004; Kline et al. 2008). In addition, there are higher numbers of nonnative species in canals than in the wetlands (Trexler et al. 2000; Gandy and Rehage unpubl. data), including some that have not yet colonized interior wetlands (Loftus et al. 2003; Kline et al. 2008).
The "Subsidized" Everglades Bass Fishery
There is a strong seasonal effect on abundance of aquatic fauna in the vicinity of canals, suggesting that canals serve as important dry-down habitats for aquatic fauna (Rehage and Trexler 2006). Canals and other artificial, deep-water habitats (culvert pools, borrow pits) provide refuge to large numbers of both native and nonnative aquatic predators in the dry season (Loftus and Kushlan 1987; Trexler et al. 2000), enhancing their survival and ultimate population sizes. Those large predatory fish populations are likely subsidized by the movement of small forage fish and shrimp into canals during the dry season (Rehage and Trexler 2006), where they fall prey to predatory fishes and alligators (Howard et al. 1995; FWC 2009). Thus, recreational fisheries in canals are likely enhanced by the combination of nutrient enrichment, movement of prey into canals during seasonal dry-downs, and the low habitat complexity of canals which may increase predator efficiency (Savino et al. 1992; Howard et al. 1995, Turner et al. 1999).
The bass fishery in Everglades canals, most notably in L-67A (FWC 2010a), may be considered a "subsidized" fishery, i.e., one that is not self-sustaining, since it depends on adjacent marshes. Unlike elsewhere in the system, WCA 3A marshes remain flooded and considerably deep even in the peak of the dry season, likely providing foraging opportunities for bass and other large-bodied taxa year-round. During the dry season, other canals become disconnected from marshes, and predators become dependent solely on prey that moved into the canals at the beginning of the dry season in addition to the in situ production, both of which may deplete over the dry season. In canals that bisect agricultural and urban areas, this prey subsidy is completely absent. Thus, canals may act as sinks for forage species produced in the wetlands, many of which become prey for the large numbers of resident and seasonal canal inhabitants (Hunt 1953; Loftus and Kushlan 1987; Howard et al. 1995; J.L. Kline, ENP, pers. comm.). The loss of forage species to canal inhabitants could affect predators that forage in the wetlands, such as wading birds; however, these effects have not yet been studied. In natural wetlands, bass are not numerous (Loftus and Kushlan 1987; Loftus and Eklund 1994), comprising only about 7% of the large-fish community (Chick et al. 2004), but are becoming more numerous in deep-water habitats or when these are readily available.
Population Sink for Alligators
Alligators, which prey on fishes but provide them with dry-season refugia in their ponds, have disappeared from former habitat in peripheral Everglades marshes because of both drainage and saltwater incursion (Mazzotti and Brandt 1994). While canals adjacent to wetlands provide habitat for dense populations of alligators, these populations are dominated by adults and exhibit negligible production compared to interior habitats (Chopp et al. 2003; Figure 8). Through much of the year, the hydrology of south Florida canals is representative of a rainfall-driven system (MacVicar 1985, Abtew et al. 2010); however, during wet-season runoff events, water levels in canals are actively manipulated, resulting in more extreme and unpredictable fluctuations than in marsh habitats (Walters and Gunderson 1994, Abtew et al. 2010). Nests of alligators built along canals are frequently flooded leading to zero recruitment of hatchling alligators in canal habitats (Chopp 2003; Figure 9).
The structure and abundance of alligator holes may also be influenced by proximity to canals; alligator holes closest to canals are more likely to have monotypic stands of cattails while those farthest from canals provide more species-rich habitats (Palmer and Mazzotti 2004). Furthermore, adult alligators that rely on canals may no longer construct and maintain alligator holes, and hence fewer alligator holes are located near canals (Kushlan 1974; Campbell and Mazzotti 2004; Palmer and Mazzotti 2004; Brandt et al. 2010; Figure 8). This shift in alligator behavior may adversely affect populations of marsh fishes, amphibians, and wading birds that rely on alligator holes for dry-season habitat. The steep banks of canals may make them unsuitable as foraging habitat by wading birds, as compared to the sloping banks of shallower alligator holes (Kushlan 1972). However, many canals in the remnant Everglades (ENP and the WCAs) have some surface water connection to the marshes and banks that are more gently sloping than those of urban canals (Rehage and Trexler 2006). With fewer alligator holes and high predation regimes in canals, fewer fishes may be available to recolonize wetlands in the wet season, possibly decreasing prey production for taxa of conservation concern, such as wading birds and alligators.
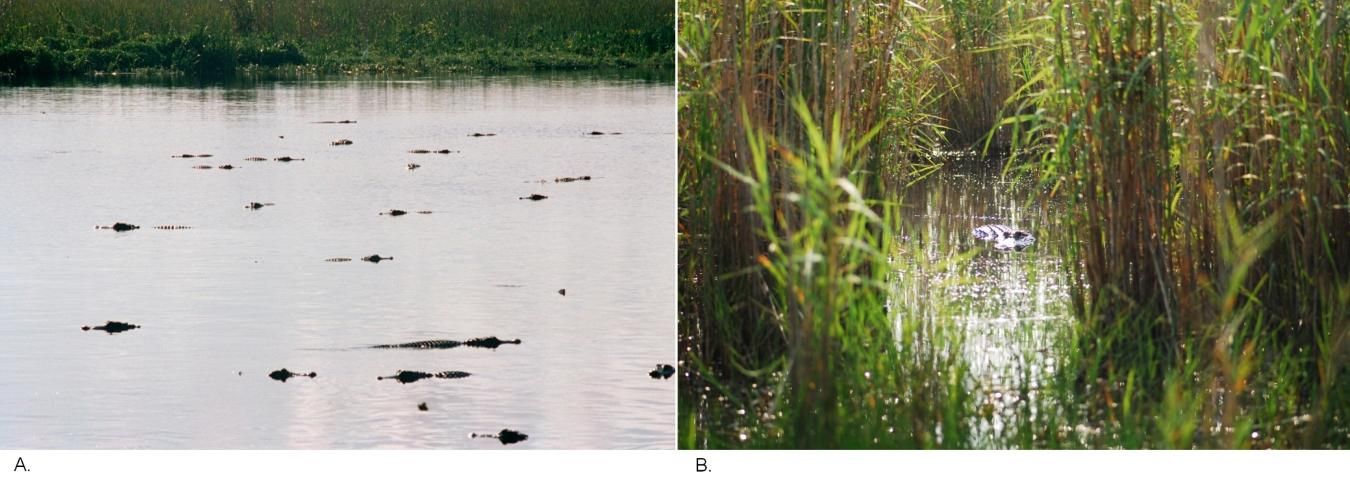
Credit: Wellington Guzman, University of Florida
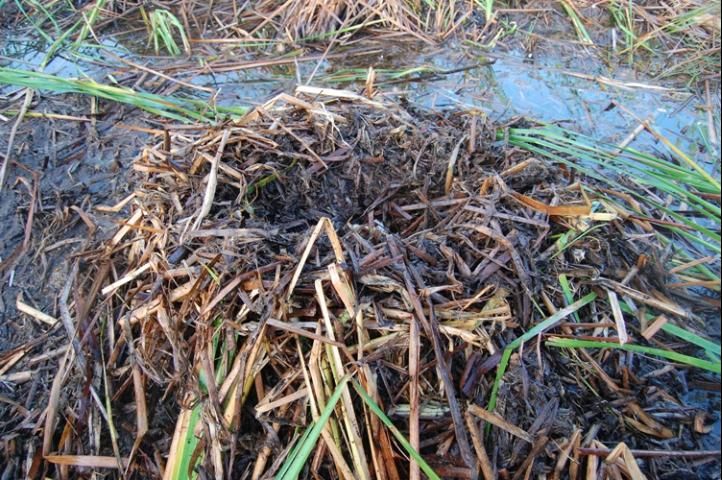
Credit: Brian Jeffery, University of Florida
Manatee Mortality
Warm water in canals during cold winters attracts manatees, where they have been known to become trapped and killed by locks and water-control gates (Ackerman et al. 1995). In fact, structure-related mortality is the second greatest human-caused mortality factor for manatees. From 1974 through 2005, 184 manatee deaths were attributed to navigation locks or water-control structures operated by the state of Florida or the USACE (FWC 2007).
Mitigating the Impacts of Everglades Canals and Levees
Canals and levees are necessary structures in south Florida's water-management system; however, as this paper demonstrates, their presence has resulted in multiple, negative ecological effects in the EPA. CERP has focused considerable attention on the effects of canals and levees in draining wetlands and impounding waters, and on the ecological effects in adjacent wetlands. The removal or modification of levees and canals in the EPA would benefit the ecosystem in several ways:
- Restore wetland sheet flow and decrease rapid canal routing of water
- Allow for the movement of particulate and dissolved materials, and the passive dispersal of native plant and animal species and their propagules across wetlands
- Reduce potential for rapid, long-distance transport of aquatic pollutants (nutrients, pesticides, etc.)
- Restore processes that support the persistence of marsh vegetation patterns and tree islands
- Increase freshwater flow to southern coastal estuaries, decrease harmful pulsed freshwater releases to northern estuaries, and reduce saltwater intrusion in coastal well fields
- Restore more natural hydropatterns to support prey of alligators and wading birds and reduce alligator nest flooding
- Reduce connectivity to urban and agricultural areas, which act as a source of nutrients, pollutants and nonnative species
- Reconnect fragmented wetlands that have been partitioned by aquatic and terrestrial barriers
- Restore functional landscape mosaic that can fulfill seasonal habitat requirements for alligators, wading birds, snail kites, and their prey
- Reduce the extent of very deep and simplified low-quality fish habitats
- Reduce the unnatural pooling of water in marshes, caused by impoundment, which will lessen the incidence of tree-island flooding and reduce the need for regulatory flood releases downstream
- Allow natural processes such as fire to move across the landscape
- Reduce genetic isolation of aquatic species
- Eliminate artificial aquatic and terrestrial habitats that support introduced plants and animals and facilitate their dispersal into the Everglades
- Restore the natural richness and abundance of native fish and invertebrates that are critical prey for wading birds
- Reduce spread of aquatic invertebrates that may affect native animals and pose health risks for humans
- Restore native apple snail populations to improve foraging success of the endangered Everglades snail kite
- Remove the thermal refugia provided for tropical nonnative fishes
- Limit spread of invasive plants and reduce management costs
Decomp: "The Heart of Everglades Restoration"
The WCA 3 Decompartmentalization and Sheet Flow Enhancement Project (Decomp) has been referred to as "the heart of Everglades restoration" (USACE and SFWMD 2002, 13). The project's goal is to restore sheet flow between WCA 3A, WCA 3B, and Shark River Slough by removing obstructions to natural flow patterns caused by canals, levees, and roads (Figure 10). Conceptual design features include backfilling all or part of the Miami Canal south of S-8 to the east coast protective levee, increasing the conveyance capacity of the North New River Canal (to compensate for that lost from the Miami Canal), elevating and bridging portions of the Tamiami Trail, and backfilling/degrading L-67A and other levee-canal complexes (L-68A, L-67C, L-29 and L-28) (USACE and SFWMD 2002; Figure 10).
The USACE and SFWMD are preparing to begin the Decomp Physical Model (DPM), a field-scale test designed to address hydrological and key ecological uncertainties prior to deciding on whether to effect permanent removal of levees and backfilling of canals (USACE and SFWMD 2010). Scheduled to begin in early 2011, the DPM is intended to increase knowledge about the potential effects of restoring sheet flow and ecosystem connectivity. The primary goals are to (1) "understand the effect of increased [flow] velocity and durations on ridge and slough biogeochemistry and (2) determine the effects of complete backfilling, partial backfilling, and no backfilling on hydrology and sediment transport" (USACE and SFWMD 2010, 13).
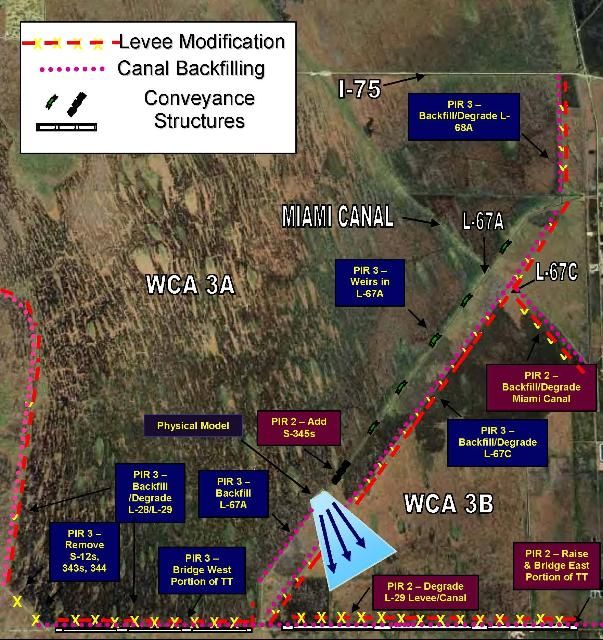
Credit: USACE and SFWMD (2010)
A Holistic Ecosystem Perspective
In addition to research specifically testing the effects of the DPM, more rigorous science is needed to understand the wide-reaching impacts of canals and levees in the EPA. A major research question focuses on how canals are used as aquatic refuges by Everglades aquatic fauna. Do canals act as sources of colonists after the dry season, as sinks for wetland production at the end of the wet season, or both for different groups of animals? The role of canals as habitats and movement corridors also demands further inquiry; do canals result in higher colonization rates of nonnative fishes into wetlands, and, if so, how far into wetlands? A comparison of community patterns and seasonal dynamics in canals that are open directly to wetlands (e.g., L-67A) with canals isolated from wetlands (e.g., L-31N) would be particularly informative. Such research would establish the degree by which populations of native and introduced predatory fishes (e.g., the productive largemouth bass fishery in Everglades canals) are sustained by resource subsidies from adjacent wetlands.
There are also social issues to be addressed, in particular how Decomp may alter recreational opportunities. Visions for the future of south Florida's water-management infrastructure must consider alternatives to meeting recreational needs without compromising restoration goals. Fishing opportunities can be maintained even if large sections of canals are backfilled. For example, the loss of bass fishing that would result from filling in L-67 might be mitigated by providing fishing opportunities in the Stormwater Treatment Areas (STAs) or other impoundments closer to urban areas. Artificial ponds, such as those created in the 1970s when the Florida Fish and Wildlife Conservation Commission constructed islands as high-water habitat for deer, are structurally similar to natural alligator holes and enhance ecological diversity of the Everglades landscape (Campbell and Mazzotti 2004). Moreover, airboat trails would continue to allow access to interior wetlands for small boats such as air boats, jon boats, and canoes. Many anglers enjoy fishing for bass in the natural wetlands. Efforts could be made to promote fishing in alligator holes, around tree islands, and in sloughs to anglers who have never tried that sport. Fishing and other recreational activities could also be promoted in more urbanized canals (and ponds) through greater accessibility, and habitat and/or stock enhancement programs.
While many of the restorative activities within CERP are directed towards correcting the impacts caused by canals and levees, some of the solutions call for construction and operation of new canals, levees, and reservoirs. CERP calls for the addition of nearly 805 km (500 miles) of new levees and canals on the periphery of the Everglades while removing more than 386 km (240 miles) of these structures from the interior (Layzer 2008). We recommend that restoration benefits from the construction of these new artificial aquatic habitats be considered with caution in light of the past ecological harm caused by canals and levees. We urge managers and engineers to avoid the adverse effects of "business as usual" by working closely with ecologists and hydrologists to give careful consideration of potential impacts across multiple ecological scales, and to devise and study new ways to deliver water in an environmentally sustainable manner. In the words of Albert Einstein, "We can't solve problems by using the same kind of thinking we used when we created them."
Acknowledgements
We thank the following individuals for reviewing drafts of this circular and providing feedback: N. Aumen, B. Bellinger, P. Frederick, J. Harvey, S. Huebner, W. Kitchens, J. Kline, R. Kobza, M. Nungesser, G. Redfield, S. Romañach, and F. Sklar. We also thank J. Kline and the South Florida Natural Resources Center for sharing the DataForEVER dataset of water temperatures in Everglades National Park; and C. McVoy and the South Florida Water Management District for providing aerial photographs of ridge and slough degradation. We are grateful to G. R. Best and USGS's Greater Everglades Priority Ecosystems Science program for supporting this project.
References
Abrahamson, W. G. and D. C. Hartnett. 1990. Chapter 5: Pine Flatwoods and Dry Prairies. Pp. 103–149. In: Ecosystems of Florida. R.L. Myers and J.J. Ewel (eds.). University of Central Florida Press, Orlando, Florida.
Abtew, W., C. Pathak, R. S. Huebner, and V. Ciuca. 2010. Chapter 2: Hydrology of the South Florida Environment. Pp. 2–1 through 2–73. In: 2010 South Florida Environmental Report. Florida Department of Environmental Protection (FDEP) and the South Florida Water Management District (SFWMD), West Palm Beach, Florida.
Ackerman, B. B., S. D. Wright, R. K. Bonde, D. K. Odell, and D. J. Banowetz. 1995. Trends and patterns in the mortality of manatees in Florida, 1974–1992. Pp. 223–258. In: Population Biology of the Florida Manatee. T.J. O'Shea, B.B. Ackerman, and H.F. Percival (eds). National Biological Service Information and Technology Report 1. Washington, D.C.
Bates, A. L., W.H. Orem, J. W. Harvey, and E. C. Spiker. 2002. Tracing sources of sulfur in the Florida Everglades. Journal of Environmental Quality 31:287–299.
Belshe, J. F. 1961. Observations on an introduced tropical fish, Belonesox belizanus, in southern Florida. M.S. Thesis. University of Miami, Coral Gables, Florida.
Bennetts, R. E., M. W. Collopy, and J. A. Rodgers, Jr. 1994. The snail kite in the Florida Everglades: a food specialist in a changing environment. Pp. 507–532. In: Everglades: the Ecosystem and its Restoration. S.M. Davis and J.C. Ogden (eds.). St. Lucie Press, Delray Beach, Florida.
Brandt, L. A., K. M. Portier, and W. M. Kitchens. 2000. Patterns of change in tree islands in Arthur R. Marshall Loxahatchee National Wildlife Refuge from 1950 to 1991. Wetlands 20:1–14.
Brandt, L. A., M. R. Campbell, and F. J. Mazzotti. 2010. Spatial distribution of alligator holes in the central Everglades. Southeastern Naturalist 9(3):487–496.
Campbell, M. R., and F. J. Mazzotti. 2004. Characterization of natural and artificial alligator holes. Southeastern Naturalist 3(4):583–594.
Carr, R., J. Zanmanillo, and J. Pepe. 2002. Archaeological profiling and radiocarbon dating of the Ortona Canal (8GL4), Glades County, Florida. Florida Anthropologist 55(1):3–24.
Carriger, J. F., G. M. Rand, P. R. Gardinali, W. B. Perry, M. S. Tompkins, A. M. Fernandez. 2006. Pesticides of potential ecological concern in sediment from south Florida canals: an ecological risk prioritization for aquatic arthropods. Soil and Sediment Contamination: An International Journal 15 (1):21–45.
Cattau, C. E., J. Martin and W. M. Kitchens. 2010. Effects of an exotic prey species on a native specialist: Example of the snail kite. Biological Conservation 143(2):513–520.
Cervone, S., J. Schardt, and B. Hassell. 2004. Types of Florida Water Bodies: Canals. In: Plant Management in Florida Waters. V. Ramey (ed.). University of Florida, Institute of Food and Agricultural Sciences, Gainesville, Florida. https://plants.ifas.ufl.edu/manage/overview-of-florida-waters/waterbody-types/canals/. Accessed August 25, 2022.
Chick, J. H., C. R. Ruetz III, and J. C. Trexler. 2004. Spatial scale and abundance patterns of large fish communities in freshwater marshes of the Florida Everglades. Wetlands 24:652–664.
Childers, D. L., R. F. Doren, R. Jones, G. B. Noe, M. Rugge, and L. J. Scinto. 2003. Decadal change in vegetation and soil phosphorus pattern across the Everglades landscape. Journal of Environmental Quality 32:344–362.
Chopp, M. D. 2003. Everglades alligator (Alligator mississippiensis) production and natural history in interior and canal habitats at Arthur R. Marshall Loxahatchee National Wildlife Refuge. M.S. Thesis. University of Florida, Gainesville, Florida.
Chopp, M. D., H. F. Percival, and K. G. Rice. 2003. Northern Everglades Canals: Alligator Population Sources or Sinks? IN U.S. Geological Survey Greater Everglades Science Program: 2002 Biennial Report (Open-File Report 03-54).
Comprehensive Everglades Restoration Plan (CERP). 2010. Why Restore the Everglades - Part 4 Ecosystem Problems Center on Water. The CERP website: http://www.evergladesplan.org/about/why_restore_pt_04.aspx
Courtenay, W. R., Jr. 1997. Nonindigenous fishes. Pp. 109–122. In: Strangers in Paradise. D.S. Simberloff, D.C. Schmitz, and T.C. Brown (eds.). Island Press, Washington, D.C.
Darby, P. C., D. J. Mellow, and M. L. Watford. 2007. Food handling difficulties for snail kites capturing non-native apple snails. Florida Field Naturalist 35(3):79–85.
Davis, S. E. and J. C. Ogden. 1994. Towards ecosystem restoration. Pp. 769–796. In: Everglades: the Ecosystem and its Restoration. S.M. Davis and J.C. Ogden (eds.). St. Lucie Press, Delray Beach, Florida.
Doren, R. F., T. V. Armentano, L.D. Whiteaker, and R.D. Jones. 1997. Marsh vegetation patterns and soil phosphorus gradients in the Everglades ecosystem. Aquatic Botany 56(2):145–163.
Ferriter, A., K. Serbesoff-King, M. Bodle, C. Goodyear, B. Doren, and K. Langeland. 2004. Chapter 8E: Exotic Species in the Everglades Protection Area. Everglades Consolidated Report, South Florida Water Management District, West Palm Beach, Florida.
Fleming D. M., W. F. Wolfe, and D. L. DeAngelis. 1994. Importance of landscape heterogeneity to wood storks in Florida Everglades. Environmental Management 18:743–757.
Flora, M. D. and P. C. Rosendahl. 1982. Historical changes in the conductivity and ionic characteristics of the source water for the Shark River Slough, Everglades National Park, Florida, USA. Hydrobiologia 97(3):249–254.
Florida Fish and Wildlife Commission (FWC). 2007. Florida manatee management plan: Trichechus manatus latirostris. Florida Fish and Wildlife Conservation Commission, Tallahassee, Florida.
Florida Fish and Wildlife Commission (FWC). 2009. The City Fisher, Issue 38. July–September 2009, South Region Fisheries Management Section, Florida Fish and Wildlife Conservation Commission, West Palm Beach, Florida.
Florida Fish and Wildlife Commission (FWC). 2010a. Florida's Top Spots for Black Bass 2010. FWC website: https://myfwc.com/fishing/freshwater/sites-forecasts/black-bass/. Accessed August 25, 2022.
Florida Fish and Wildlife Commission (FWC). 2010b. Everglades & Francis S. Taylor WMA. FWC website: https://myfwc.com/recreation/lead/everglades/. Accessed August 25, 2022.
Forman, R. T. T. and L. E. Alexander. 1998. Roads and their major ecological impacts. Annual Review of Ecology and Systematics 29:207–231.
Fourqurean, J. W., R. D. Jones, and J. C. Zieman. 1993. Process influencing water column nutrient characteristics and phosphorus limitation of phytoplankton biomass in Florida bay, FL, USA: inferences from spatial distributions. Estuarine, Coastal and Shelf Science 36(3):195–314.
Frederick, P. C., D. Axelrad, T. Atkeson, and C. Pollman. 2005. Contaminants research and policy: The Everglades mercury story. National Wetlands Newsletter 27:3–6.
Frederick, P. C. and N. U. A. Jayasena. In press. Altered pairing behaviour and reproductive success in White Ibises exposed to environmentally relevant concentrations of methylmercury. Proceedings of the Royal Society B: Biological Sciences.
Fuller, P. L., L. G. Nico, and J. D. Williams. 1999. Nonindigenous Fishes. Introduction into Inland Waters of the United States. American Fisheries Society Special Publication 27, Bethesda, Maryland, 613 pp.
Gaiser, E. E., L. J. Scinto, J. H. Richards, K. Jayachandran, D. L. Childers, J. C. Trexler, and R. D. Jones. 2004. Phosphorus in periphytonmats provides the best metric for detecting low-level P enrichment in an oligotrophic wetland. Water Research 38:507–516.
Gaiser, E. E., J. C. Trexler, J. H. Richards, D. L. Childers, D. Lee, A. L. Edwards, L. J. Scinto, K. Jayachandrana, and R. D. Jones. 2005. Cascading ecological effects of low-level phosphorus enrichment in the Florida Everglades. Journal of Environmental Quality 34:717–723.
Grimshaw, H. J., R. G. Wetzel, M. Brandenburg, K. Segerblom, L. J. Wenkert, G. A. Marsh, W. Charnetzky, J. E. Haky, and C. Carraher. 1997. Shading of periphyton communities by wetland emergent macrophytes: decoupling of algal photosynthesis from microbial nutrient retention. Archiv fuer Hydrobiologie 139(1):17–27.
Gunderson, L. H. and W. F. Loftus. 1993. The Everglades. Pp. 199–255. In: Biodiversity of the Southeastern United States. W.H. Martin, S.G. Boyce, and A.C. Echternacht (eds.). John Wiley and Sons, New York.
Harvey J. W., S. L. Krupa, and J. M. Krest. 2004. Ground-water recharge and discharge in the central Everglades. Ground Water 42:1090–1102.
Harvey, J. W., and P. V. McCormick. 2009. Groundwater's significance to changing hydrology, water chemistry, and biological communities of a floodplain ecosystem, Everglades, South Florida, USA. Hydrogeology Journal 17:185–201.
Hoffman, W., G. T. Bancroft, and R. J. Sawicki. 1994. Foraging habitat of wading birds in the water conservation areas of the Everglades. Pp. 585–614. In: Everglades: the Ecosystem and its Restoration. S.M. Davis and J.C. Ogden (eds.). St. Lucie Press, Delray Beach, Florida.
Hofmockel, K. 1999. Effects of hydrologic management decisions on marsh structure in Water Conservation Area 2A of the Everglades, Florida. M.S. Thesis, Duke University, Durham, North Carolina.
Hollingsworth, R. G. and R. H. Cowie. 2006. Apple snails as disease vectors. Pp 121–132. In: Global Advances in Ecology and Management of Golden Apple Snails. R.C. Joshi and L.S. Sebastian (eds.). Philippine Rice Research Institute, Nueva Ecija, Philippines.
Howard, K. S., W. F. Loftus, and J. C. Trexler. 1995. Seasonal dynamics of fishes in artificial culvert pools in the C-111 basin, Dade County, Florida. Final Report to the U. S Army Corps of Engineers as Everglades N. P. Cooperative Agreement #CA5280-2-9024.
Hunt, B. P. 1953. Food relationship between Florida spotted gar and other organisms in the Tamiami Canal, Dade County, Florida. Transactions of the American Fisheries Society 82:14–32.
Johnson, K. 2009. Freshwater angler surveys. Florida Fish and Wildlife Conservation Commission, Fish and Wildlife Research Institute. FWC Website: http://research.myfwc.com/features/view_article.asp?id=34099.
Kline, J. L., W. F. Loftus, K. Kotun, Z. W. Fratto, M. Robinson, S. Perry, and J. C. Trexler. 2008. Fish introductions into Everglades wetlands: an unforeseen consequence of restoration? Greater Everglades Ecosystem Restoration: Planning Policy and Science Meeting. Naples, Florida. July 28–August 1, 2008.
Kobza, R. M., J. C. Trexler, W. F. Loftus, and S. Perry. 2004. Community structure of fishes inhabiting aquatic refuges in a threatened Karst wetland and its implications for ecosystem management. Biological Conservation 116:153–165.
Krupa S, S. Hill, and S. Diaz. 2002. Investigation of Surface Water-Groundwater Interactions at S-7 Pump Station, Broward and Palm Beach Counties, Florida. Technical Publication WS-11, South Florida Water Management District, West Palm Beach, Florida.
Kushlan, J. A. 1974. Observations of the role of the American alligator in the southern Florida wetlands. Copeia 993–996.
Larsen, L. G. and J. W. Harvey. 2010. How vegetation and sediment transport feedbacks drive landscape change in the Everglades and wetlands worldwide. The American Naturalist 176(3): E-Article.
Larsen, L. G., J. W. Harvey, and J. P. Crimaldi. 2007. A delicate balance: ecohydrological feedbacks governing landscape morphology in a lotic peatland. Ecological Monographs 77(4):591–614.
Layzer, J. A. 2008. Natural Experiments: Ecosystem-Based Management and the Environment. MIT Press, Cambridge, Massachusetts.
Light, S. S. and J. W. Dineen. 1994. Water control in the Everglades: A historical perspective. Pp. 47–84. In: Everglades: the Ecosystem and its Restoration. S.M. Davis and J.C. Ogden (eds.). St. Lucie Press, Delray Beach, Florida.
Lodge, T. E. 2010. The Everglades Handbook: Understanding the Ecosystem. 3rd Edition. CRC Press, Boca Raton, Florida.
Loftus, W. F. and A. Eklund. 1994. Long-term dynamics of an Everglades small-fish assemblage. Pp. 461–483. In: Everglades: the Ecosystem and its Restoration. S.M. Davis and J.C. Ogden (eds.). St. Lucie Press, Delray Beach, Florida.
Loftus, W.F. and J.A. Kushlan. 1987. Freshwater fishes of southern Florida. Bulletin of the Florida State Museum, Biological Sciences 31:147–344.
Loftus, W. F., L. G. Nico, J. Kline, S. A. Perry, and J. C. Trexler. 2003. Recent Fish Introductions into Southern Florida Freshwaters, with implications for the Greater Everglades region. 2nd Greater Everglades Ecosystem Restoration (GEER) Conf., April 2003, Palm Harbor, Florida. USGS Open-File Report 03-54.
Loftus, W. F., G. Ellis, M. Zokan, and J. Lorenz. 2004. Inventory of freshwater fish species within the Big Cypress National Preserve: the basis for a long-term sampling program. US Geological Survey Fact Sheet 2004-3131.
Loftus, W. F., J.C. Trexler, K. Dunker, S. E. Liston, and J. S. Rehage. 2006. Introduced Fishes in Short-Hydroperiod Wetlands: Evaluation of Sampling, Status, and Potential Effects. Final Report to the National Park Service, Everglades National Park, under CESI IA F5284-04-0039.
Lorenz, J. J. 2000. The impact of water management on Roseate Spoonbills and their piscine prey in the coastal wetlands of Florida Bay. Ph.D. Dissertation. University of Miami, Coral Gables, Florida.
MacVicar, T. K. 1985. A Wet Season Field Test of Experimental Water Deliveries to Northeast Shark River Slough. Technical Publication 85-3. South Florida Water Management District, Resource Planning Department and Resource Operations Department.
MacMahon, D. A. and W. H. Marquardt. 2004. The Calusa and their Legacy: South Florida People and their Environments. University Press of Florida, Gainesville, Florida.
Mazzotti, F. J. and L. A. Brandt. 1994. Ecology of the American alligator in a seasonally fluctuating environment. Pp. 485–505. In: Everglades: the Ecosystem and its Restoration. S.M. Davis and J.C. Ogden (eds.). St. Lucie Press, Delray Beach, Florida.
McCormick, P. V. and J. A. Laing. 2003. Effects of increased phosphorus loading on dissolved oxygen in a subtropical wetland, the Florida Everglades. Wetlands Ecology and Management 11:99–216.
McCormick, P. V., P. S. Rawlik, K. Lurding, E. P. Smith, and F. H. Sklar. 1996. Periphyton-water quality relationships along a nutrient gradient in the northern Everglades. Journal of the North American Bethological Society 15(4):433–449.
McCormick, P. V., S. Newman, S. Miao, D. E. Gawlik, D. Marley, K. R. Reddy, and T. D. Fontaine. 2002. Effects of anthropogenic phosphorus inputs on the Everglades. Pp. 83–126. In: The Everglades, Florida Bay, and Coral Reefs of the Florida Keys: An Ecosystem Sourcebook. J.W. Porter and K.G. Porter (eds.). CRC Press, Boca Raton, Florida.
McCormick, P. V., R. B. E. Shuford III, and P. S. Rawlik. 2004. Changes in macroinvertebrate community structure and function along a phosporus gradient in the Florida Everglades. Hydrobiologia 529:113–132.
McElroy, T. C., K. L. Kandl, J. Garcia, and J. C. Trexler. 2003. Extinction-colonization dynamics structure genetic variation of spotted sunfish (Lepomis punctatus) in the Florida Everglades. Molecular Ecology 12(2):355–368.
McIvor, C. C., J. A. Ley, and R. D. Bjork. 1994. Changes in freshwater inflow from the Everglades to Florida Bay including effects on biota and biotic processes: A review. Pp. 117–146. In: Everglades: the Ecosystem and its Restoration. S. M. Davis and J. C. Ogden (eds.). St. Lucie Press, Delray Beach, Florida.
National Park Service (NPS). 2005. An Assessment of the Interim Operational Plan. South Florida Natural Resources Center, Everglades National Park. http://www.npshistory.com/publications/ever/sfnrc/2005-2.pdf. Accessed August 25, 2022.
National Park Service (NPS). 2010. Everglades Archaeological Heritage. The NPS website: https://home.nps.gov/ever/learn/historyculture/archaeological-heritage.htm
Noe, G. B., D. L. Childers, and R. D. Jones. 2001. Phosphorus biogeochemistry and the impact of phosphorus enrichment: why is the Everglades so unique? Ecosystems 4:603–624
Nuttle, W. K., J. W. Fourqurean, B. J. Cosby, J. C. Zieman, and M. B. Robblee. 2000. Influence of net freshwater supply on salinity in Florida Bay. Water Resources Research 36(7):1805–1822.
Ogden, J. C. 2005. Everglades ridge and slough conceptual ecological model. Wetlands 25:810–820.
Palmer, M. L. and F. J. Mazzotti. 2004. Structure of Everglades alligator holes. Wetlands 24(1):115–122.
Patterson, K. and R. Finck. 1999. Tree islands of the WCA3 aerial photointerpretation and trend analysis project summary report. Geonex Corporation, Report to the South Florida Water Management District, St. Petersburg, Florida.
Pfeuffer, R. J and J. M. Rand. 2004. South Florida ambient pesticide monitoring program. Ecotoxicology 13:195–205.
Rawlings, T. A., K. A. Hayes, R. H. Cowie, and T. M. Collins. 2007. The identity, distribution, and impacts of non-native apple snails in the continental United States. BMC Evolutionary Biology 7:97.
Rehage, J. S. and J. C. Trexler. 2006. Assessing the net effect of anthropogenic disturbance on aquatic communities in wetlands: community structure relative to distance from canals. Hydrobiologia 569:359–373.
Rehage, J. S., K. Dunlop, and W. F. Loftus. 2009. Mosquitofish antipredator responses to non-native cichlids: a test of the prey naiveté hypothesis. Ethology 115:1–11.
Rutchey, K., T. Schall, and F. Sklar. 2008. Development of vegetation maps for assessing Everglades restoration progress. Wetlands 28(3):806–816.
Scheidt, D., J. Stober, R. Jones, and K. Thornton. 2000. South Florida Ecosystem Assessment: Everglades Water Management, Soil Loss, Eutrophication and Habitat. Report No. 904-R-00-003, United States Environmental Protection Agency, West Palm Beach, Florida.
Schofield, P. J., W. F. Loftus, R. M. Kobza, M. I. Cook, and D. H. Slone. 2010. Tolerance of nonindigenous cichlid fishes (Cichlasoma urophthalmus, Hemichromis letourneuxi) to low temperature: laboratory and field experiments in south Florida. Biological Invasions 12: 2441–2457.
Schmitz, D. C., J. D. Schardt, A. J. Leslie, F. A. Dray, J. A. Osborne, and B. V. Nelson. 1993. The ecological impact and management history of three invasive alien aquatic species in Florida. Pp. 173–194. In: Biological Pollution: The Control and Impact of Invasive Exotic Species. B. N. McKnight (ed.). Indiana Academy of Science, Indianapolis, Indiana.
Schuler, L. J. and G. M. Rand. 2008. Aquatic risk assessment of herbicides in freshwater ecosystems of south Florida. Archives of Environmental Contamination and Toxicology 54:571–583.
Science Coordination Team (SCT). 2003. The role of flow in the Everglades ridge and slough landscape. South Florida Ecosystem Restoration Working Group. http://www.sfrestore.org/sct/docs/SCT%20Flow%20Paper%20-%20Final.pdf
Scott, G. I., M. H. Fulton, E. F. Wirth, G. T. Chandler, P. B. Key, J. W. Daugomah, D. Bearden, K. W. Chung, E. D. Strozier, M. Delorenzo, S. Sivertsen, A. Dias, M. Sanders, J. M. Macauley, L. R. Goodman, M. W. Lacroix, G. W. Thayer, And J. Kucklick. 2002. Toxicological studies in tropical ecosystems: an ecotoxicological risk assessment of pesticide runoff in south Florida estuarine ecosystems. Journal of Agricultural and Food Chemistry 50:4400–4408.
Serafy, J. E., K. C. Lindeman, T. E. Hopkins, and J. S. Ault. 1997. Effects of freshwater canal discharge on fish assemblages in a subtropical bay: field and laboratory observations. Marine Ecology Progress Series 160:161–172.
Shafland, P. L. 1995. Introduction and establishment of a successful butterfly peacock fishery in southeast Florida canals. American Fisheries Society Symposium 15:443–451.
Shafland, P. L. 1996. Exotic fish assessments: An alternative view. Reviews in Fisheries Science 4(2):123–132.
Shafland, P. L. and J. M. Pestrak. 1982. Lower lethal temperatures for fourteen non-native fishes in Florida. Environmental Biology of Fishes 7(2):149–156.
Shafland, P. L., K. B. Gestring, and M. S. Stanford. 2008. Florida's exotic freshwater fishes—2007. Florida Scientist 3:220–245.
Sklar, F. and J. A. Browder. 1998. Coastal environmental impacts brought about by alterations to freshwater flow in the Gulf of Mexico. Environmental Management 22(4):547–562.
Sklar, F. and A. Van der Valk (eds.). 2002. Tree Islands of the Everglades. Kluwer Academic Publishers, Norwell, Massachusetts.
Sklar, F., C. McVoy, R. VanZee, D. E. Gawlik, K. Tarboton, D. Rudnick, and S. Miao. 2002. The effects of altered hydrology on the ecology of the Everglades. Pp. 39–82. In: The Everglades, Florida Bay, and Coral Reefs of the Florida Keys: An Ecosystem Sourcebook. J.W. Porter and K.G. Porter (eds.). CRC Press, Boca Raton, Florida.
Sonenshein, R. S. 1996. Delineation of Saltwater Intrusion in the Biscayne Aquifer, Eastern Dade County, Florida, 1995. US Geological Survey, Miami, Florida, Water-Resources Investigations Report 96-4285.
South Florida Natural Resources Center (SFNRC). 2010. DataForEVER Dataset, Everglades National Park, Homestead, FL, Generated by Jeff Kline, using Appaserver software (https://appahost.com/appaserver.html), Sacramento, CA, Public URL not currently available, please send data requests to EVER_data_request@nps.gov.
Stober, Q. J., R. D. Jones, and D. J. Scheidt. 1995. Ultra trace level mercury in the Everglades ecosystem, a multi-media canal pilot study. Water, Air, and Soil Pollution 80: 991–1001.
Surratt, D. D., M. Waldon, M. C. Harwell, and N. G. Aumen. 2008. Time-series and spatial tracking of polluted canal water intrusion into wetlands of a national wildlife refuge in Florida, USA. Wetlands 28:176–183.
Trexler, J. C., W. F. Loftus, F. Jordan, J. J. Lorenz, J. H. Chick, and R. M. Kobza. 2000. Empirical assessment of fish introductions in a subtropical wetland: an evaluation of contrasting views. Biological Invasions 2:265–277.
Trexler, J. C., W. F. Loftus, F. Jordan, J. H. Chick, K. L. Kandl, T. C. McElroy, and O. L. Bass, Jr. 2002. Ecological scale and its implications for freshwater fishes in the Florida Everglades. Pp. 153–181. In: The Everglades, Florida Bay, and Coral Reefs of the Florida Keys: An Ecosystem Sourcebook. J.W. Porter and K.G. Porter (eds.). CRC Press, Boca Raton, Florida.
Trexler, J. C., W. F. Loftus, and S. A. Perry. 2005. Disturbance frequency and community structure in a twenty-five year intervention study. Oecologia 145:140–152.
Turner, A. M., J. C. Trexler, F. Jordan, S. J. Slack, P. Geddes, and W. Loftus. 1999. Targeting ecosystem features for conservation: standing crops in the Florida Everglades. Conservation Biology 13:898–911.
U.S. Army Corps of Engineers (USACE) and South Florida Water Management District (SFWMD). 2002. Project Management Plan: WCA-3 Decompartmentalization and Sheet flow Enhancement Part 1.
U.S. Army Corps of Engineers (USACE) and South Florida Water Management District (SFWMD). 2010. Decomp Physical Model Environmental Assessment (EA) & Design Test Documentation Report (DTDR). Final, April 2010. EA Appendix E: Decomp Physical Model Science Plan.
Walters, C. J. and L. H. Gunderson. 1994. A screening of water policy alternatives for ecological restoration in the Everglades. Pp. 757–767. In: Everglades: the Ecosystem and its Restoration. S. M. Davis and J. C. Ogden (eds.). St. Lucie Press, Delray Beach, Florida.
Wanless, H. R. and B. M. Vlaswinkel. 2005. Coastal landscape and channel evolution affecting critical habitats at Cape Sable, Everglades National Park, Florida. Final Report to Everglades National Park. National Park Service. U.S. Department of Interior, https://parkplanning.nps.gov/document.cfm?documentID=71736. Accessed August 25, 2022.
Watts, D. L., M. J. Cohen, J. B. Heffernan, and T. Z. Osborne. 2010. Hydrologic modification and the loss of self-organized patterning in the ridge–slough mosaic of the Everglades. Ecosystems 13(6):813–827.
Wingard, G. L., J. B. Murray, W. B. Schill, and E. C. Phillips. 2008. Red-Rimmed Melania (Melanoides tuberculatus)—A Snail in Biscayne National Park, Florida—Harmful Invader or Just a Nuisance? U.S. Geological Survey Fact Sheet 2008-3006, 6 pp.