The objective of this publication is to provide basic information about the role of micronutrients in warm-season grass production systems in Florida. Because adequate soil fertility management is an integral component of sustainable forage management, the information presented in this publication should be of interest to stakeholders, students, and scientists interested in enhancing the productivity of pasture systems in Florida.
Warm-season grasses are vital to livestock production systems and dominate ground cover in tropical and subtropical areas. However, because most soils in humid-tropical and subtropical areas are highly weathered and often possess acidic pH and low levels of essential nutrients, proper soil fertility management is a key aspect of successful warm-season grass production. In Florida, Spodosols are the predominant soil type used for forage production. Despite their relatively deep rooting systems and efficient nutrient utilization, many popular warm-season grasses, such as bahiagrass and bermudagrass, are generally grown on low-fertility soils and are subject to periods of drought, excess water, and intensive grazing. In areas such as these, the removal of nutrients from the system due to animal consumption, harvest, and leaching can lead to nutrient imbalances in the soils, which can impact forage production and sustainability.
Micronutrients are essential elements that are required in smaller quantities than macronutrients but are equally important for proper plant growth and performance (St. John et al. 2013). An element is considered essential for plant growth if a plant fails to complete its life cycle in the absence of the element; the element's action is specific and cannot be completely replaced by another element; the element has a direct effect on the organism, or if it is a constituent of a molecule that is known to be essential (Epstein 1971). There are currently eight recognized micronutrients: boron (B), chlorine (Cl), copper (Cu), iron (Fe), manganese (Mn), molybdenum (Mo), nickel (Ni), and zinc (Zn). Below is a brief description of essential micronutrient functions in the plant.
Boron
Boron is arguably one of the least understood micronutrients involved in plant growth (Yermiyahu et al. 2001; Marschner 1995). It participates in a wide variety of processes within the plant, including sugar transport, cell wall synthesis, lignification, carbohydrate metabolism, respiration, and membrane formation (Parr and Loughman 1983; Blevins and Lukaszewski 1998; Ahmad et al. 2009). In cell wall structure, boron is involved with the movement of calcium, leading to cell wall rigidity (Blevins and Lukaszewski 1998); some research estimates that 90% of total boron in plants is in the cell wall (Loomis and Durst 1992). In root tips, new leaves, or actively growing plant regions, boron impacts meristematic tissue and ensures adequate growth (Blevins and Lukaszewski 1998).
Boron requirements vary widely by plant species, with grasses usually requiring less in comparison to other species (Marschner 1995). Deficiencies generally occur on calcareous soils with a high pH and high clay content (Marschner 1995). Symptoms of boron deficiency are more prevalent in broadleaf plants and legumes than in grasses, and may include internode shortening, chlorosis of the leaves, and malformed leaves (St. John et al. 2013). Because of the large role boron plays in plant reproduction, deficiencies can also lead to stunted growing points and dropped flowers or fruit (St. John et al. 2013). There is a very narrow gap between sufficient levels and toxic levels of boron (Marschner 1995), and inducing toxicity can be aggravated by using irrigation water that is high in boron (Carrow et al. 2001). Chlorosis along the leaf tips and leaf margins is the most common visual indicator of boron toxicity (St. John et al. 2013; Brady and Weil 2007).
Chlorine
The main functions of Cl in plants are enzyme regulation and the control of water movement in the plant (White and Broadly 2001; Brady and Weil 2007). This element also helps enhance the function of manganese in photosynthesis (St. John et al. 2013) and aids in the regulation of cell turgor pressure helping the plant maintain structure (White and Broadly 2001). Chlorine is also involved in pH regulation (White and Broadly 2001).
Chlorine deficiency results in substantially reduced plant growth, and visual signs include reduced leaf growth, leaf wilting, chlorosis, bronzing and necrosis of leaves, and reduced root growth (Brady and Weil 2007; White and Broadly 2001). Chlorine deficiency is rarely reported in turfgrasses because chlorine concentrations are generally very high in soils (St. John et al. 2013; White and Broadly 2001). High levels of chlorine that lead to toxicity generally happen in saline soils with poor drainage; chlorine is highly mobile in soil, so it easily follows the water table (White and Broadly 2001). Plant species vary in their ability to withstand chlorine toxicities, but warm-season grasses generally have a higher tolerance than cool-season grasses (St. John et al. 2013). Toxicities can be identified as plants begin to show burnt leaf tips (especially on younger leaves) and dead root tips (Brady and Weil 2007).
Copper
Copper is a transition metal essential to many plant physiological processes. It has many purposes due to the multiple chemical forms in which it can occur (Yruela 2005). It can act as a structural element in regulatory proteins and can participate in photosynthetic electron transport, mitochondrial respiration, cell wall metabolism, and hormone signaling (Marschner 1995). Copper also serves as a cofactor in many enzymes, especially those involved in the conversion of amino acids to proteins (St. John et al. 2013), which are important to proper plant growth and development (Brady and Weil 2007; Yruela 2005). Copper is vital in the formation of lignin, an important structural component of plant cells (Marschner 1995).
Copper deficiency is uncommon but can occur in calcareous soils or soils high in organic matter, such as the muck soils found in south Florida (St. John et al. 2013). Due to the immobility of copper in the plant, symptoms of deficiency are first observed in the younger leaves and include wilting due to insufficient lignin production and distortion and yellowing of the leaves (St. John et al. 2013). Copper toxicity is more common due to the multiple chemical forms of the element (Yruela 2005). Mining, manufacturing, and agriculture can release excess amounts of copper into the environment, increasing the chances of copper toxicity (Yruela 2005). Toxicity symptoms generally appear as stunted root growth and chlorosis of the leaves with a red coloration on the leaf margins (Brady and Weil 2007; Yruela 2005).
Iron
Iron is considered the most important essential micronutrient in plants because of the roles it plays in plant growth and metabolism (Rout and Sahoo 2015). The majority of iron found in plants, roughly 80%, is associated with photosynthetic cells (such as chlorophyll) and the electron transport system (Briat et al. 2007). Molecules dependent on iron are found in photosystem II, photosystem I, the ferredoxin molecule, and the cytochrome (Varotto et al. 2002), indicating the large impact iron has on photosynthesis and, consequently, on overall plant performance (Briat et al. 2007). In plant metabolism, iron is an important component of proteins and enzymes, having a large impact on photosynthesis, chlorophyll synthesis, respiration, nitrogen fixation, uptake mechanisms, hormone synthesis, and DNA synthesis (Kim and Reese 1992; St. John et al. 2013).
Iron is predominately immobile in plants, so deficiency symptoms will be observed in newer growth first (St. John et al. 2013). Yellowing of the leaves is a common sign of chlorosis, or insufficient chlorophyll production. This correlates with iron deficiency because of the importance of iron to proper chlorophyll production (Hochmuth 2011). Other nutrients, such as phosphorus, copper, manganese, and zinc, can inhibit iron uptake by the plant (Ghasemi-Fasaei and Ronaghi 2008). Iron toxicity takes places mainly on submerged soils and leads to dark discolorations of the leaf margins and dark, slimy roots (Brady and Weil 2007).
Manganese
Manganese is the second most important micronutrient to plants and is crucial for its roles in photosynthesis, enzyme activation, root growth, root pathogen resistance, and nitrogen assimilation (St. John et al. 2013). In photosynthesis, manganese participates in the assimilation of photosynthetic proteins and enzymes (Millaleo et al. 2010). Manganese is associated with a minimum of 35 enzymes in plants and functions as a cofactor with several of these enzymes in the biosynthesis of lignin (St. John et al. 2013). A high lignin content in grasses is associated with disease resistance (St. John et al. 2013).
Manganese toxicity is most common in low (less than 4.8) pH soils (Marschner 1995) or soils that are waterlogged (Hernandez-Soriano et al. 2012). Toxicity is characterized by a reduction in biomass and photosynthesis (Millaleo et al. 2010). Manganese accumulates in plant tissues and alters processes such as enzyme activity, absorption, translocation and utilization of other mineral elements (Ducic and Polle 2005), further decreasing plant growth. Manganese deficiency can appear at low and high soil pH and lead to competition with other nutrients that aggravate additional toxicities (St. John et al. 2013). Since manganese is less mobile in plants, younger leaves are the first to show symptoms of deficiency, which is characterized by interveinal yellowing (chlorosis) due to the sensitivity of chloroplast to manganese (St. John et al. 2013; Brady and Weil 2007).
Molybdenum
Molybdenum is a micronutrient metal required for plant growth and, similar to other metals, is predominantly important for enzymes (Kaiser et al. 2005), especially those associated with nitrogen fixation and nitrogen assimilation (Brady and Weil 2007; Marschner 1995). Due to the specific types of enzymes it is involved with, molybdenum is essential for converting nitrate to amino acids and inorganic phosphorus to organic forms (St. John et al. 2013). The plant requirement for molybdenum is very small, with only the micronutrient nickel being needed in smaller amounts (St. John et al. 2013).
Molybdenum deficiency is rare because of the small amount required by plants, but can occur in plants grown on sandy, acidic soils in humid regions (St. John et al. 2013). Because of the role molybdenum plays in plant nitrogen fixation, visual symptoms are similar to those of nitrogen deficiency (St. John et al. 2013; Brady and Weil 2007). Visual signs of molybdenum deficiency vary by plant species, but may include leaf mottling and cupping, flaccid leaves, and necrotic leaf tips (Kaiser et al. 2005). Toxicity symptoms are also very rare (St. John et al. 2013) but may occur in areas where biosolids are utilized as a fertilizer (McBride et al. 2004). Visual signs include leaf malformation and a golden-yellow discoloration of the shoot tissue (Marschner 1995).
Nickel
The most recent nutrient to be identified as essential for plant growth is nickel (St. John et al. 2013; Liu et al. 2011; Bai et al. 2006). Nickel has important roles in several aspects of plant growth, and predominantly acts as a component of seven key enzymes involved in nitrogen metabolism and biological nitrogen fixation (Ahmad and Ashraf 2011; Liu et al. 2011). Urease is one of the most important enzymes because of the role it plays in nitrogen metabolism in plants (Liu et al. 2011; St. John et al. 2013). Nickel acts as a cofactor to enable the enzyme urease to catalyze the conversion of urea into the ammonium ion, which can then be used as a source of nitrogen by plants (Liu et al. 2011; Marschner 1995). Urea conversion is not possible without the presence of nickel (Liu et al. 2011).
While nickel deficiency is rarely identified and usually occurs in soils with a pH > 6.7 (Liu et al. 2011), visual indicators include leaf tip necrosis, delayed nodulation, chlorosis in young leaves, and stunted leaves (Liu et al. 2011; Brady and Weil 2007). In a literature review by St. John et al. (2013), it was concluded that no viable data existed for nickel deficiency in grasses. In contrast, nickel toxicity is well documented and becoming more of a problem because of increased pollution from mining, the burning of fossil fuels, vehicle emissions, waste disposal, agricultural fertilizer application, and application of organic fertilizers such as biosolids (Ahmed and Ashraf 2011; St. John et al. 2013). Toxicity can be identified by reduced seed germination, reduced shoot or root growth, leaf spotting, reduced plant growth, and abnormal flower shape (Brady and Weil 2007; Ahmad and Ashraf 2011).
Zinc
The most important role that Zinc (Zn) plays in plants is to lower activation energies and increase the rates of chemical reactions as a catalyst (St. John et al. 2013). In addition, zinc has a structural role in enzymes (St. John et al. 2013). Growth hormone production, starch formation, and seed maturation and production are also impacted by zinc (Brady and Weil 2007). Zinc affects protein molecules that control DNA replication and gene expression (Coleman 1992). Specific to warm-season grasses, zinc aids in the maintenance of photosynthesis and fungal resistance (Hull 2001).
Zinc deficiency symptoms vary among plant species (St. John et al. 2013). Burnell et al. (1990) suggested that warm-season grasses are more sensitive to low levels of zinc than cool-season grasses. Visual symptoms of zinc deficiency in grasses include shriveling and darkening of younger leaves (Brady and Weil 2007). Zinc toxicity can also be problematic to plants; again, symptoms and quantities vary by plant species. Sartain (1996) identified the tolerance level of bermudagrass to elevated zinc levels to be very high. Visual signs of zinc toxicity include shortened roots and chlorotic younger leaves (St. John et al. 2013).
Forage Responses to Micronutrient Fertilization
Crop responses to micronutrient fertilization are generally influenced by several factors, such as soil micronutrient concentration, pH, plant species, and fertility management. More specifically for forages, response to micronutrients is often less predictable than forage responses to N, P, and K fertilization.
Although most soils in Florida often exhibit low levels of micronutrients, fertilization studies have not always been conclusive. Positive impacts of micronutrient fertilization on forage responses have been rarely reported in Florida. This is mainly due to the fact that the mechanisms involved in micronutrient dynamics in the soil are complex and are also influenced by management and environmental conditions. In addition, predicting micronutrient availability also represents a major challenge. Although soil and plant tissue analyses are important diagnostic tools to predict forage responses to macronutrients, micronutrient availability varies significantly (spatially and temporarily). Factors such as soil temperature, moisture conditions, and interaction with other nutrients strongly affect micronutrient availability and the extent to which crops will likely respond to micronutrient fertilization.
Soil pH is generally the most important soil property determinant of micronutrient availability. Availability of boron, copper, iron, manganese, and zinc decreases as soil pH increases (Figure 1). Micronutrient deficiencies are generally more common in calcareous or over-limed soils. Soil texture and organic matter concentrations also affect micronutrient availability.
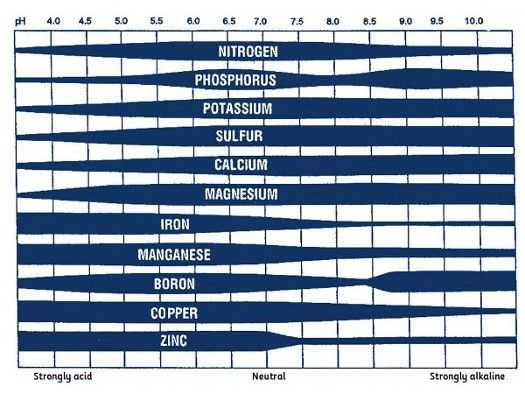
Credit: Maria Silveira
Management conditions such as nitrogen fertilization and forage utilization (haying vs. grazing) also affect soil micronutrient concentrations and the likelihood that crops will respond to micronutrient fertilization. Warm-season grasses produced in tropical and subtropical areas are more likely subjected to grazing than mechanical harvesting. Pastures in these areas receive limited or no inorganic fertilizer inputs, and therefore depend heavily on the recycling of nutrients in the animal-plant-soil system. Animal excreta is an important source of nutrients, as an estimated 85% of consumed nutrients are returned to the pasture via animal excreta (Vendramini 2013). Although the distribution of animal excreta is often not uniform, it represents an important source of micronutrients in grazed systems.
Repeated mechanical harvests of forage increases the likelihood of micronutrient deficiency due to the removal of micronutrients from the system. Therefore, frequent soil and tissue testing to monitor micronutrient concentration in fields dedicated to hay and silage is recommended.
Critical levels of micronutrients in soil and plant tissue have not been determined for forage crops in Florida; however, information presented in Table 1 illustrates the average values commonly reported in the literature. In many instances, the range between toxic and deficient levels is very narrow, so micronutrients should not be used indiscriminately. Supplemental application of micronutrient fertilizers are generally not needed or recommended for forage production in Florida. Although symptoms of micronutrient deficiency may occur, micronutrient fertilization rarely results in positive impacts on forage production. For instance, iron deficiency occurs quite frequently in Florida during spring, but the symptoms tend to disappear as the growing season progresses. Research indicated no benefit of iron fertilization to bahiagrass pastures.
Summary
Although essential micronutrients are important for forage production in Florida, research data indicated application of supplemental micronutrient-containing fertilizer to low-input grazing systems is not needed or recommended. In general, soil pH is the major determinant of micronutrient availability. Soil and tissue testing represent effective diagnostic tools to help producers monitor pasture soil fertility status. When soil pH is maintained within the adequate range for the specific forage crop, micronutrient availability is not expected to limit forage production and nutritive value.
References
Ahmad, M.S., and M. Ashraf. 2011. "Essential roles and hazardous effects of nickel in plants." Reviews of Environmental Contamination and Toxicology. 214: 125–167.
Bai, C., C.C. Reilly, and B.W. Wood. 2006. "Nickel deficiency disrupts metabolism of ureides, amino acids, and organic acids of young pecan foliage." Plant Physiology. 140:433–443.
Ball, D.M., C.S. Hoveland, and G.D. Lacefield. 1996. Southern Forages. 2nd ed. Atlanta, Georgia: Potash and Phosphate Institute and the Foundation for Agronomic Research.
Blevins, D.G., and K.M. Lukaszewski. 1998. "Boron in plant structure and function." Annual Review of Plant Physiology and Plant Molecular Biology. 49:481–500.
Brady, N.C. and R.C. Weil. 2007. The Nature and Properties of Soils. 14th ed. revised. New Jersey: Prentice Hall.
Briat, JF, C. Curie, and F. Gaymard. 2007. "Iron utilization and metabolism in plants." Current Opinion in Plant Biology. 10:276–282.
Burnell, J.N., I. Suzuki, and T. Sugiyama. 1990. "Light induction and the effect of nitrogen status upon the activity of carbonic anhydrase in maize leaves." Plant Physiology. 94:384–387.
Carrow, R.N., D.V. Waddington, and P.E. Rieke. 2001. Turfgrass soil fertility and chemical problems: Assessment and management. Chelsea, Michigan: Ann Arbor Press.
Coleman, J.E. 1992. "Zinc Proteins: Enzymes, storage proteins, transcription factors, and replication proteins." Annual Review of Biochemistry. 61:897–946.
Ducic, T., and A. Polle. 2005. "Transport and detoxification of manganese and copper in plants." Brazilian Journal of Plant Physiology. 17:103–112.
Dubeux, J.C.B., Jr., L.E. Sollenberger, J.M.B. Vendramini, R.L. Stewart, Jr., and S.M. Interrante. 2006. "Litter mass, deposition rate, and chemical composition in bahiagrass pastures managed at different intensities." Crop Science. 46: 1299–1304
Dubeux, J.C.B., L.E. Sollenberger, B.W. Mathews, J.M. Scholberg, and H.Q. Santos. 2007. "Nutrient cycling in warm-climate grasslands." Crop Science. 47:915–928.
Epstein, E. Mineral Nutrition of Plants. Principles and Perspectives. 1971. New York, London, Sydney, Toronto: John Wiley and Sons, Inc.
Ghasemi-Fasaei, R., and A. Ronaghi. 2008. "Interaction of iron with copper, zinc, and manganese in wheat as affected by iron and manganese in a calcareous soil." Journal of Plant Nutrition. 31:839–848.
Hernandez-Soriano, M.C., F. Degryse, E. Lombi, E. Smolders. 2012. "Manganese toxicity in barley is controlled by solution manganese and soil manganese speciation." Soil Science Society of America Journal. 76: 399–407.
Hochmuth, G. 2011. Iron (Fe) Nutrition in Plants. SL353. Gainesville: University of Florida Institute of Food and Agricultural Sciences. https://edis.ifas.ufl.edu/ss555
Hull, R.J. 2001. "Zinc used by turfgrass." Turfgrass Trends. 10:7–11.
Kaiser, B.N., K.L. Gridley, J.N. Brady, T. Phillips, and S.D. Tyerman. 2005. "The role of Molybdenum in agricultural plant production." Annals of Botany. 96:745–754.
Kim, J., and D.C. Rees. 1992. "Structural models for the metal centers in the nitrogenous molybdenum-iron protein." Science. 257: 1677–82.
Liu, G., E.H. Simonne, and Y. Li. 2011. Nickel Nutrition in Plants. HS1191. Gainesville: University of Florida Institute of Food and Agricultural Sciences. https://edis.ifas.ufl.edu/hs1191
Loomis, W.D., and R.W. Durst. 1992. "Chemistry and biology of boron." Bio Factors. 4:229–239.
Marschner, H. 1995. Mineral nutrition of higher plants. 2nd ed. London: Academic Press.
Mathews, B.W., S.C. Miyasaka, and J.P. Tritschler. 2004. "Mineral nutrition of C4 forages grasses." In Warm-season (C4) grasses, ed. Moser, L.E. et. al., 217–265. Madison, WI: ASA, CSSA, and SSSA.
McBride, M.B., B.K. Richards, and T. Steenhuis. 2004. "Bioavailability and crop uptake of trace elements in soil columns amended with sewage sludge products." Plant Soil. 262:71–84.
Millaleo, R., M. Reyes-Diaz, A. Ivanov, M.L. Mora, and M. Alberdi. 2010. "Manganese as essential and toxic element for plants: transport, accumulation and resistance mechanisms." Journal of Soil Science and Plant Nutrition. 10:470–481.
Parr, A.J., and B.C. Loughman. "Boron and membrane functions in plants," in Metals and Micronutrients: Uptake and Utilization by Plants (London: Academic Press, 1983), 87–107.
Rout, G.R., and S. Sahoo. 2015. "Role of Iron in Plant Growth and Metabolism." Reviews in Agriculture Science. 3:1–24.
Sartain, J.B. 1986. Interaction effects of P and Zn nutrition on the growth of bermudagrass. Paper presented at: ASA, CSSA, and SSSA Annual Meetings, New Orleans, LA. 30 Nov.–5 Dec. 1986. Agronomy Abstracts, 78:138
Silveira, M.L. "Soil acidity and its relationship with nutrient use efficiency." Department of Soil and Water Science Program, UF/IFAS Range Cattle Research & Education Center, 2013, http://sfbfp.ifas.ufl.edu/articles/article_2013_february.shtml.
Silveira, M.L., F.M. Rouquette, Jr., G.R. Smith, H.M.S. da Silva, and J.C.B Dubeux, Jr. 2014. "Soil-fertility principles for warm-season perennial forages and sustainable pasture production." doi:10.2134/FG-2013-0041-RV.
St. John, R.A., N.E. Christians, H. Liu, and N.A. Menchyk. 2013. "Secondary nutrients and micronutrient fertilization," in Turfgrass: Biology, use, and management. 521–541.
Varotto, C., D. Maiwald, P. Pesaresi, P. Jahns, F. Salamini, and D. Leister. 2002. "The metal ion transporter IRT1 is necessary for iron homeostasis and efficient photosynthesis in Arabidopsis thaliana." Plant Journal. 31:589–599.
Vendramini, J. 2013. "The importance of micronutrient fertilization of warm-season grass pastures in Florida." The Florida Cattleman and Livestock Journal. 77(12): 38–42
White, P.J., and M.R. Broadley. 2001. "Chloride in soils and its uptake and movement within the plant: a review." Annals of Botany. 88: 967–988.
Wright, D.L., and E.B. Whitty. 2011. Fertilization of Agronomic Crops. SS-AGR-152. Gainesville: University of Florida Institute of Food and Agricultural Sciences. https://edis.ifas.ufl.edu/aa130
Yermiyahu, U., R. Keren, and Y. Chen. 2001. "Effect of composted organic matter on boron uptake by plants." Soil Science Society of America Journal. 65:1436–1441.
Yruela, I. 2005. "Copper in Plants." Brazilian Journal of Plant Physiology. 17:145–156.