Introduction
This publication provides an overview of Rift Valley fever virus (RVFV) history, geographic distribution, vectors, hosts, epidemiology, and control, and is intended to deliver important information about RVFV to public health professions, veterinarians, researchers, stakeholders in mosquito control, and the general public.
Rift Valley fever (RVF) is an acute, febrile illness that poses a serious global threat to the health of humans and livestock. This illness is caused by the Rift Valley fever virus (RVFV), an RNA virus belonging to the Phlebovirus genus in the family Phenuiviridae and order Bunyavirales (Abudurexiti et al. 2019). The first isolation of the virus was from the Great Rift Valley of Kenya in 1930 during an epidemic among sheep, and since that time the geographic range of RVFV has been largely confined to the African continent (Davies 2010). In 2000, however, RVFV spread to the Arabian Peninsula and caused two major outbreaks in Yemen and Saudi Arabia, marking the first emergence of RVFV outside its traditional geographic range (Madani et al. 2003). Despite the restricted prevalence of RVFV in Africa and the Arabian Peninsula, the movement of viremic hosts, such as domestic livestock or humans, either by international trade or migration and the widespread presence of putatively competent vectors may facilitate the eventual emergence and spread of RVFV in previously unaffected countries, including the United States (Rolin et al. 2013). Outbreaks of RVFV among livestock are associated with severe illness, mass abortion, and death, especially in sheep, cattle, and goats, which often leads to significant economic losses and trade restrictions in affected counties. In humans, RVFV infections typically result in mild, self-limiting febrile symptoms but can progress to more severe illnesses, including encephalitis, blindness, hepatitis, and hemorrhagic fever, which can be fatal (Ikegami and Makino 2011).
Although RVFV is primarily transmitted to humans and livestock by arthropod vectors, mainly mosquitoes, the virus can also spread through other routes of transmission, including direct contact with infectious blood, tissue, or fluids, or inhalation of aerosolized virus particles (Bales et al. 2012; Rolin et al. 2013; Baba et al. 2016; Linthicum et al. 2016). While there have been no reports of human-to-human transmission, the aerosol dissemination of RVFV has been well documented, making this agent a potential biological weapon (Smithburn et al. 1949; Sidwell and Smee 2003; Rolin et al. 2013). Because numerous mosquito species across several genera can transmit the virus and because of its potential devastating consequences for agriculture and public health, the Centers for Disease Control and Prevention and National Institute of Allergy and Infectious Diseases have classified RVFV as an “overlap select agent” and a “Category A priority pathogen” in the United States (Rolin et al. 2013). These classifications are reserved for pathogens that present the greatest danger to health and safety.
RVFV Distribution
Rift Valley fever virus has a broad geographic distribution across Africa with enzootic and epizootic cases reported throughout much of the continent. However, the frequency and distribution of reported outbreaks have increased, particularly since 2000, when the virus spread to the Arabian Peninsula (CDC 2020a). Multiple epizootics among domestic animals occurred between 2000 and 2020 (Figure 1), including in Senegal, Mauritania, and the Gambia in West Africa between 2012 and 2016; in South Africa and Namibia beginning in 2009; and in Angola in 2016. Niger, a country that is part of the Sahel and located in northern Central Africa, also had a moderate RVF epizootic in 2016 (CDC 2020a). In 2006 and 2007, a large epizootic occurred in Kenya, Somalia, and Tanzania in East Africa, following heavy rains associated with El Niño Southern Oscillation (ENSO) climatic conditions (Lutomiah et al. 2014; Linthicum et al. 2016). In 2018, another epizootic occurred in Kenya, also following unusually heavy rains, this time during the long rainy season with human cases confirmed in June (WHO 2018). In 2016, a multi-year epizootic began in Uganda and was the first laboratory-confirmed detection in this country in 48 years (Shoemaker et al. 2019). In 2019, multiple human and animal cases were detected on the Island of Mayotte off the coast of East Africa in the Indian Ocean (Youssouf et al. 2020).
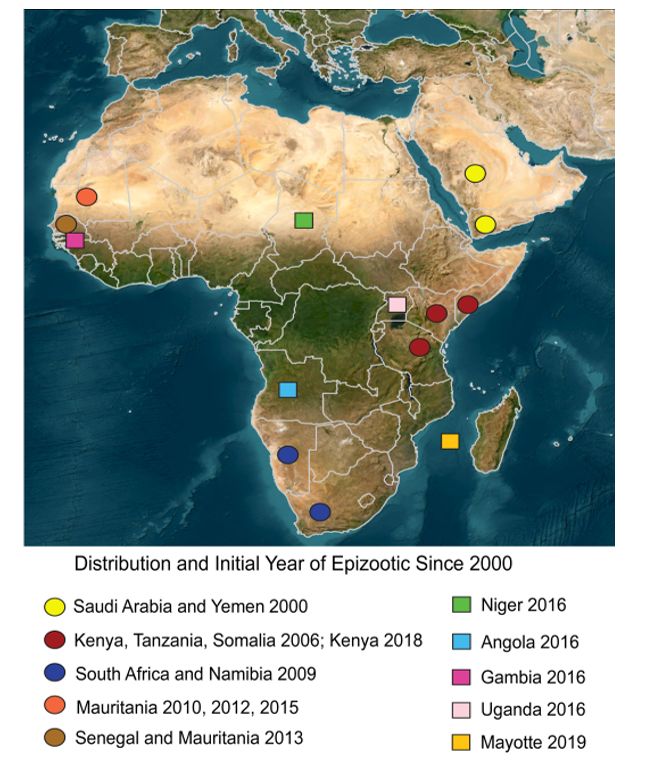
Credit: Lindsay P. Campbell, UF/IFAS
The large number of mosquitoes capable of transmitting RVFV, the diversity of susceptible vertebrate hosts, and the distribution of the virus across a broad range of bioclimatic conditions make understanding its potential establishment and spread into new geographic areas a global priority (Chevalier et al. 2010; Rolin et al. 2013; Gibson et al. 2022). Establishment in new geographic areas could occur through the introduction of viremic ruminants, including domestic livestock, which could then infect competent mosquito vectors in the new region. This scenario has been hypothesized as the means of introduction from Africa to the Arabian Peninsula (Chevalier et al. 2010; Rolin et al. 2013). Other potential routes of establishment have been deemed to be relatively low risk but not impossible, including the movement of infected mosquito vectors into a new geographic area through trade or travel (Rolin et al. 2013). There is also limited evidence that infected humans traveling to a non-endemic location could play a role in RVFV emergence by serving as an infectious reservoir and triggering an outbreak (Golnar et al. 2014; Gibson et al. 2022). However, although RVFV amplification in humans to mosquito-transmissible levels has been documented (Meegan 1979), in areas where RVFV is established, human hosts likely play a negligible role in transmission cycles compared to other mammalian hosts (Rolin et al. 2013). Nevertheless, the travel of infectious humans should not be ignored as a potential route of RVFV introduction and emergence. The risk of establishment through intentional introduction from a bioweapon remains unknown (Rolin et al. 2013), but it is certainly a concern for the United States and other non-endemic countries with conditions conducive to a sustained transmission cycle.
RVFV Vectors and Vertebrate Hosts
Diverse mosquito species belonging to at least six genera have been found present during RVFV outbreaks, and naturally infected with the virus in various portions of Africa (Linthicum et al. 2016). Most isolations of RVFV from mosquitoes have been from females of the genus Aedes, a biologically diverse, species-rich genus comprising roughly 1,270 species and approximately 75 subgenera worldwide (Wilkerson et al. 2015). Approximately three quarters of African Aedes species from which RVFV has been isolated are considered floodwater mosquitoes, a biologically convergent group that lays eggs in shallow ground depressions that will become pools of water after heavy rainfall or flooding. Important subgenera of floodwater mosquitoes implicated in RVFV transmission include Aedimorphus, Catageiomyia, Neomelaniconion, and Ochlerotatus. In particular, several species of the subgenus Neomelaniconion have been found naturally infected with RVFV in multiple countries on the African continent, suggesting that they could play a role as vectors of the virus. Aedes (Neomelaniconion) circumluteolus has been found infected with RVFV in Kenya, South Africa, and Uganda, and Aedes (Neomelaniconion) mcintoshi has been found infected with RVFV in Zimbabwe, South Africa, and Kenya (Linthicum et al. 2016).
Other genera of mosquitoes from which RVFV has been isolated, indicating natural infection with the virus, include Anopheles, Coquillettidia, Culex, Eretmapodites, and Mansonia (Linthicum et al. 2016). Most Culex species from which RVFV has been isolated are from the large subgenus Culex, including Culex antennatus, Culex pipiens complex members, Culex theileri, and Culex zombaensis. Several species of Anopheles, from the subgenera Anopheles and Cellia, have been found naturally infected with RVFV. Notably, Anopheles (Anopheles) coustani and Anopheles (Cellia) squamosus have been found repeatedly infected with RVFV in multiple countries. Other mosquito species from which RVFV has been isolated include Coquillettidia fuscopennata, Coquillettidia grandidieri, Eretmapodites chrysogaster, Eretmapodites intermedius, Eretmapodites quinquevittatus, Mansonia africana, and Mansonia uniformis. In addition to mosquitoes, RVFV has infrequently been isolated from blackflies (genus Simulium) and ticks (genus Amblyomma).
Other criteria that must be satisfied to incriminate vector species include vector competence (the ability of a species to transmit the virus between infectious and susceptible hosts, determined in laboratory studies) and contact between the suspected vector and vertebrate hosts (often determined through blood meal analysis). Vector competence and host associations have been examined for a few of the species found naturally infected with RVFV.
Many of the mosquito species that have been found naturally infected with RVFV have also been found to naturally feed upon large mammals during outbreaks. During an RVFV outbreak in Kenya (1982–83) cattle were found to be the major source of bloodmeals for Aedes (Neomelaniconion) lineatopennis (85.6%), Aedes (Aedimorphus) dentatus (90.7%), Aedes (Aedimorphus) cumminsii (79.2), Ae. sudanensis (92.3%), Aedes circumluteolus (67.5%), Aedes (Aedimorphus) quasiunivittatus (66%), and Culex antennatus (93.3%). Other vertebrate hosts identified included human, canine, giraffe, hare, and horse or zebra (Linthicum, Kaburia et al. 1985). During a subsequent outbreak (2006–07) in Kenya, Aedes (Aedimorphus) ochraceus and Aedes mcintoshi fed mainly on goats (35.2%–37.6%), cattle (15.3%–16.4%), donkeys (10.7%–12.7%), sheep (5.9%–6.3%), and humans (5.15.3%) (Lutomiah et al. 2014). These studies indicate that many species implicated in RVFV through virus detection also feed upon potential amplifying hosts.
Through laboratory studies, researchers can determine whether putative vector species that feed upon amplifying hosts and that have been found infected with a pathogen can biologically transmit between infected and uninfected hosts. Relatively few species of African mosquitoes have been examined for vector competence in detail. Turrell et al. (1996) evaluated the vector competence of Aedes caspius, Culex pipiens, Culex antennatus, Culex perexiguus, Culex poicilipes, and Anopheles pharoensis from Egypt. Overall transmission rates were 20% for Aedes caspius, 7% for Culex pipiens, 7% for Culex antennatus, and 11% for Culex perexiguus. In a subsequent study, Turrell et al. (2008) examined eight African species for vector competence of RVFV. Aedes palpalis, Aedes mcintoshi, Aedes circumluteolus, Culex antennatus, and Culex pipiens transmitted RVFV by bite after oral exposure while Ae. calceatus, Ae. aegypti and Cx. quinquefasciatus did not. Jupp and Cornel (1988) examined several species from South Africa and found that Aedes unidentatus, Culex poicilipes , and Aedes argenteopunctatus transmitted RVFV by bite. Using an east-African strain of RVFV and west-African mosquitoes, Ndiaye et al. (2016) found that Aedes vexans, Culex quinquefasciatus , and Culex poicilipes were capable of transmitting the virus.
Ungulates are considered the natural vertebrate hosts of RVFV. Sheep, cattle, and goats are the primary vertebrate hosts in RVFV epidemiology, although wild ungulates are also frequently infected with the virus, especially during epizootics (Britch et al. 2013; Linthicum et al. 2016). During and immediately following the widespread and temporally protracted East African epizootic of RVFV (2006–2007), relatively high rates of exposure (antibody prevalence) were observed in African buffalo (Syncerus caffer), common warthog (Phacochoerus africanus), giraffe (Giraffa camelopardalis), and domesticated camel (Camelus dromedarius) (Britch et al. 2013; Linthicum et al. 2016). Birds, nonhuman primates, and rodents are also likely to be exposed to RVFV through the bites of infectious vectors (Linthicum et al. 2016); however, these vertebrates are not thought to be important amplification hosts of the virus.
RVFV Transmission Cycles
Transmission cycles of RVFV are heavily influenced by vector ecology and rainfall since flooding is required to stimulate egg hatching of the floodwater Aedes species that serve as primary vectors. Low-lying areas in savanna grasslands are the most important sites for vector mosquito production (Davies et al. 1985; Linthicum et al. 1985a). These areas are called dambos in East Africa and pans or vleis in South Africa and range dramatically in size from a few meters to multiple kilometers (Linthicum et al. 2016). A key aspect of RVFV cycles is that selected species of floodwater Aedes may transmit RVFV vertically from mother to egg (transovarial transmission; Linthicum et al. 1985b). Eggs infected with RVFV that are produced toward the end of the rainy season are oviposited on soil or vegetation in dry but flood-prone areas because rainfall is highly seasonal. These eggs may remain dormant and viable for months to years until stimulated to hatch by a flood, leading to RVFV-infected adults capable of transmission when seeking their first blood meal (Linthicumet al. 1985b) (Figure 2).
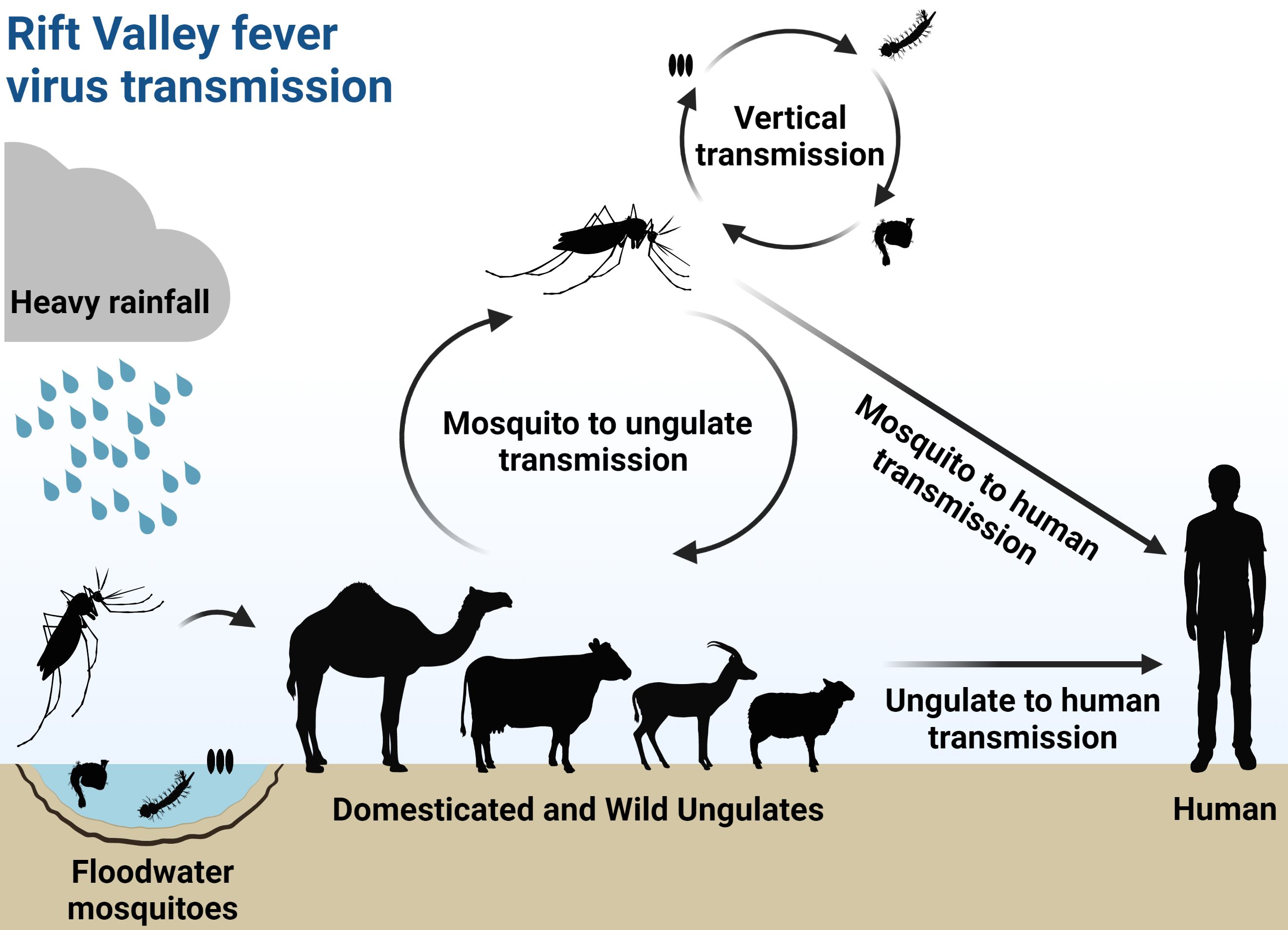
Credit: Abdullah A. Alomar, UF/IFAS
There are two types of transmission cycles for RVFV, the enzootic cycle, and the epizootic/epidemic cycle. The enzootic cycle occurs more frequently and is the “maintenance cycle” that enables virus persistence in the environment (Linthicum et al. 2016). This cycle is characterized by limited RVFV transmission among a small number of animal hosts, such as domestic or wild ungulates that are driven by floodwater Aedes mosquitoes. Although this cycle results in little to no impact on animal or human health, it maintains the virus in the environment by replenishing the bank of RVFV-infected mosquito eggs in the soil (Linthicum et al. 1985b). Therefore, in years with low to moderate amounts of rainfall and flooding, horizontal transmission of RVFV is low and often goes unnoticed (Linthicum et al. 2016).
In contrast, in years with sustained, excessive rainfall and long periods of flooding, epizootic/epidemic cycles of RVFV transmission may occur, leading to an increase in veterinary cases compared to the enzootic cycle (i.e., an epizootic) and oftentimes an outbreak in the human population (i.e., an epidemic) (Linthicum et al. 2016). This phenomenon has been well documented and in the Horn of Africa is now associated with El Niño-Southern Oscillation (ENSO) events, during which elevated surface temperatures in the eastern equatorial Pacific Ocean and western equatorial Indian Ocean lead to prolonged and very heavy rainfall (Linthicum et al. 1999). In such instances, RVFV transmission begins as a normal enzootic cycle but transitions to an epizootic/epidemic cycle as the disease ecology underlying the system changes. Excessive rain raises the water table, increasing both the extent and duration of flooding, as well as the amount of emergent vegetation. These conditions are highly favorable to mosquito production and survival (Linthicum et al. 1983; 1984), and as a consequence, the number of RVFV-infected Aedes eggs that hatch is much greater than in normal years, leading to higher RVF incidence in animal hosts and subsequent RVFV amplification (Linthicum et al. 2007). As floodwaters persist, they become stagnant and attractive to oviposition by additional mosquito species capable of transmitting RVFV, particularly those in the genera Culex, Mansonia, and Coquillettidia described above (Linthicum et al. 1983; 1984). The initial infections are undoubtedly driven by floodwater Aedes, but once these secondary vector species emerge, they perpetuate transmission and spread RVFV horizontally to susceptible hosts throughout the area, domestic livestock in particular (Logan et al. 1991; Lutomiah et al. 2014). Large-scale amplification of RVFV in livestock is the hallmark of an epizootic, which can have devastating consequences on herds of cattle, sheep, and goats. Importantly, some of the vectors driving the epizootic event are opportunistic in feeding and will bite a variety of mammalian hosts, including humans (Lutomiah et al. 2014). Such vectors in combination with human activities that include drinking unpasteurized milk, handling or consumption of infected blood or meat, or handling aborted fetuses, can bridge transmission to the human population from the epizootic cycle, leading to an RVF epidemic. Although RVFV can cause a severe human illness, and humans can develop viral titers sufficient to infect vector mosquitoes (Meegan 1979), humans are not considered to be important amplifying hosts for the virus, neither during epizootics nor in epidemics.
In addition to vector-borne transmission, RVFV can be transmitted to humans through direct contact with infected livestock or through aerosols when an animal is slaughtered or butchered (Linthicum et al. 2016). In instances of airborne transmission, aerosolized virus particles are inhaled, so there is a real risk of infection for people in close proximity to sick or dead animals, even in the absence of contact. The segment of the population at greatest risk of infection during RVFV epizootic/epidemic are those who work closely with live animals (e.g., farmers, shepherds), who work as butchers or in slaughterhouses, and who provide veterinary care for the animals (Linthicum et al. 2016).
RVFV Epidemiology and Control
Epidemiology
Rift Valley fever virus was first identified in 1930 during a rainy season in the Rift Valley province in Kenya (Daubney and Hudson 1931). After the first detection in Kenya, RVFV caused several epizootics and epidemics in many African countries, including Egypt, Somalia, Zimbabwe, Tanzania, South Africa, Senegal, Sudan, Nigeria, Mauritania, and Madagascar (El Akkad 1978; Meegan 1979; Saluzzo et al. 1987; Zeller et al. 1997; Gerdes 2004). Impacts on agriculture and human health were elevated during these events. For example, in 1951, RVFV caused a serious epizootic in South Africa with an estimated 100,000 sheep dead and half a million aborted (Weiss 1957). An extensive epizootic and human epidemic of RVF in 1977 in Egypt resulted in a substantial number of mortalities and abortions in sheep, cattle, and camels and deaths among humans (Meegan 1979; Ahmed Kamal 2011). In September 2000, RVFV emerged for the first time outside the African continent on the Arabian Peninsula, leading to hemorrhagic fever cases and fatalities in humans and major losses in livestock populations in Saudi Arabia and Yemen (Abdo-Salem et al. 2006; Madani et al. 2003). Recently, countries in the Mediterranean region including Tunisia and Turkey have reported RVFV seropositivity in sheep and cattle, indicating previous exposure to the virus and highlighting the potential risk of RVFV emergence in new locations (Zouaghi et al. 2021; Kwaśnik et al. 2021).
Clinical Manifestations
Livestock infected with RVFV may experience a sudden onset of fever, weakness, vomiting, nasal discharge, diarrhea, reduction in milk production, abortion, or high rates of severe illness and death (Figure 3). The consequences of infection are age-dependent, and young livestock are more likely to develop a severe form of the illness than adult animals (Davies et al. 1985; Kwaśnik et al. 2021). The severity of illness also varies among host species, as sheep are the animals most severely affected among domestic livestock followed by goats, cattle, camels, and water buffaloes (in decreasing order) (Mariner 2018). The incubation period for acute cases in sheep and goats can be fewer than 24 hours, with death occurring in 1–3 days. In infected flocks of sheep, close to 100% of animals show symptoms, with mortality rates varying by age from as high as 95% in newborn lambs to 5%–30% in adults (Mariner 2018). In cattle, mortality rates may reach as high as 30% in newborns, while calves that survive RVF may suffer debilitating disease with hepatitis and jaundice that persists for months. Adult cattle usually survive the infection, as mortality rates in infected herds tend to be less than 5%. Despite high survival, morbidity rates can be high with adult animals showing many of the symptoms described above, especially pregnancy loss in cows (Mariner 2018).
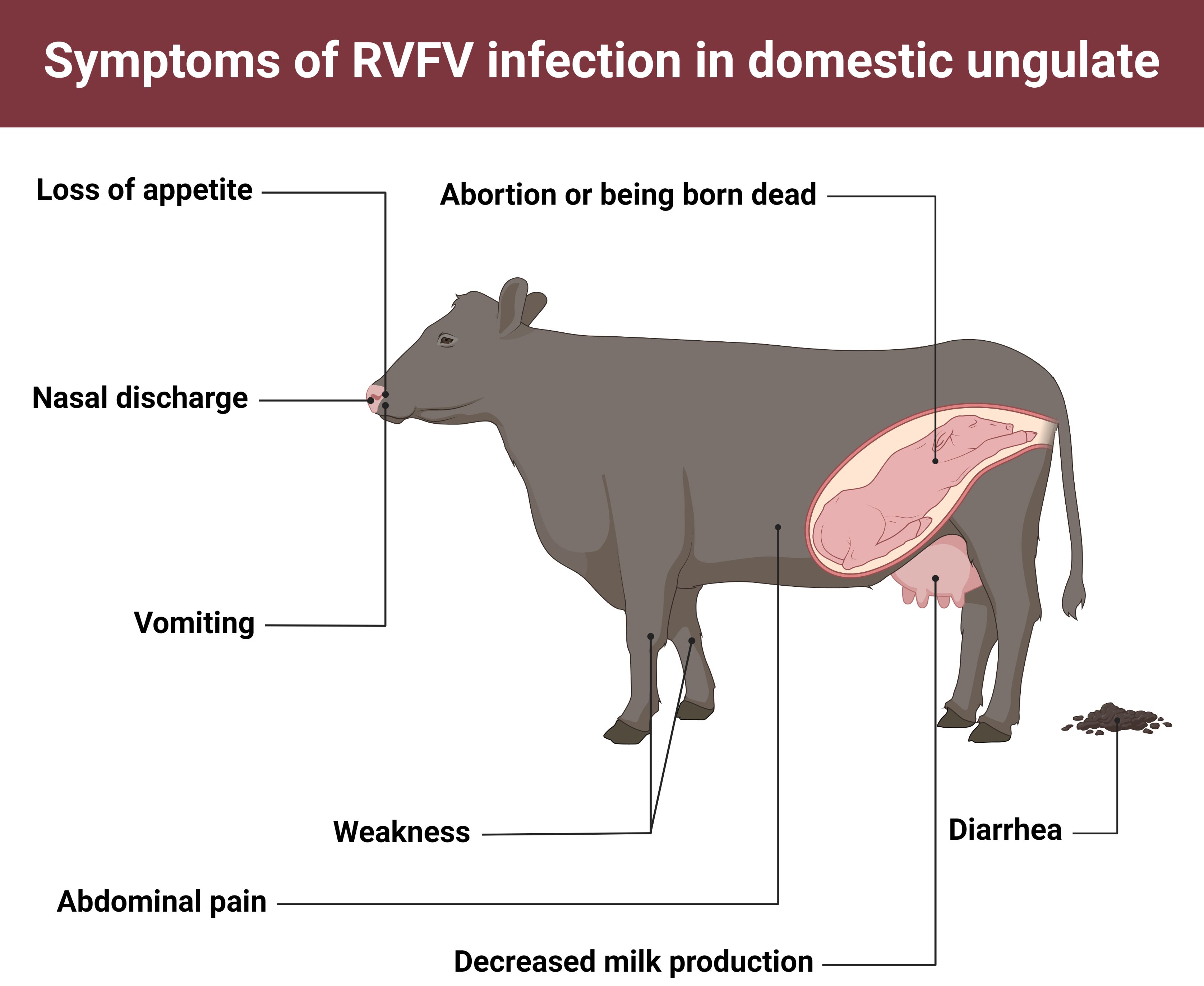
Credit: Abdullah A. Alomar, UF/IFAS
In humans, the RVFV incubation period is typically 2–6 days following exposure (CDC 2020b). Infections are often asymptomatic or result in a self-limiting febrile illness (e.g., headache, fever, weakness, dizziness, muscle pain, rigor, and joint pain) that lasts a few days. In contrast, 8%–10% of human infections develop into a severe illness that can have a range of clinical manifestations, including encephalitis (inflammation of the brain), retinitis (ocular disease), hepatitis, and hemorrhagic fever (Figure 4). The case-fatality ratio in humans is low in comparison to livestock, but of the severe manifestations, fatality is most often associated with hemorrhagic fever. Although less than 1% of human cases lead to hemorrhagic fever, approximately 50% of these are fatal, with death usually occurring only 3–6 days after the onset of symptoms (CDC 2020b).
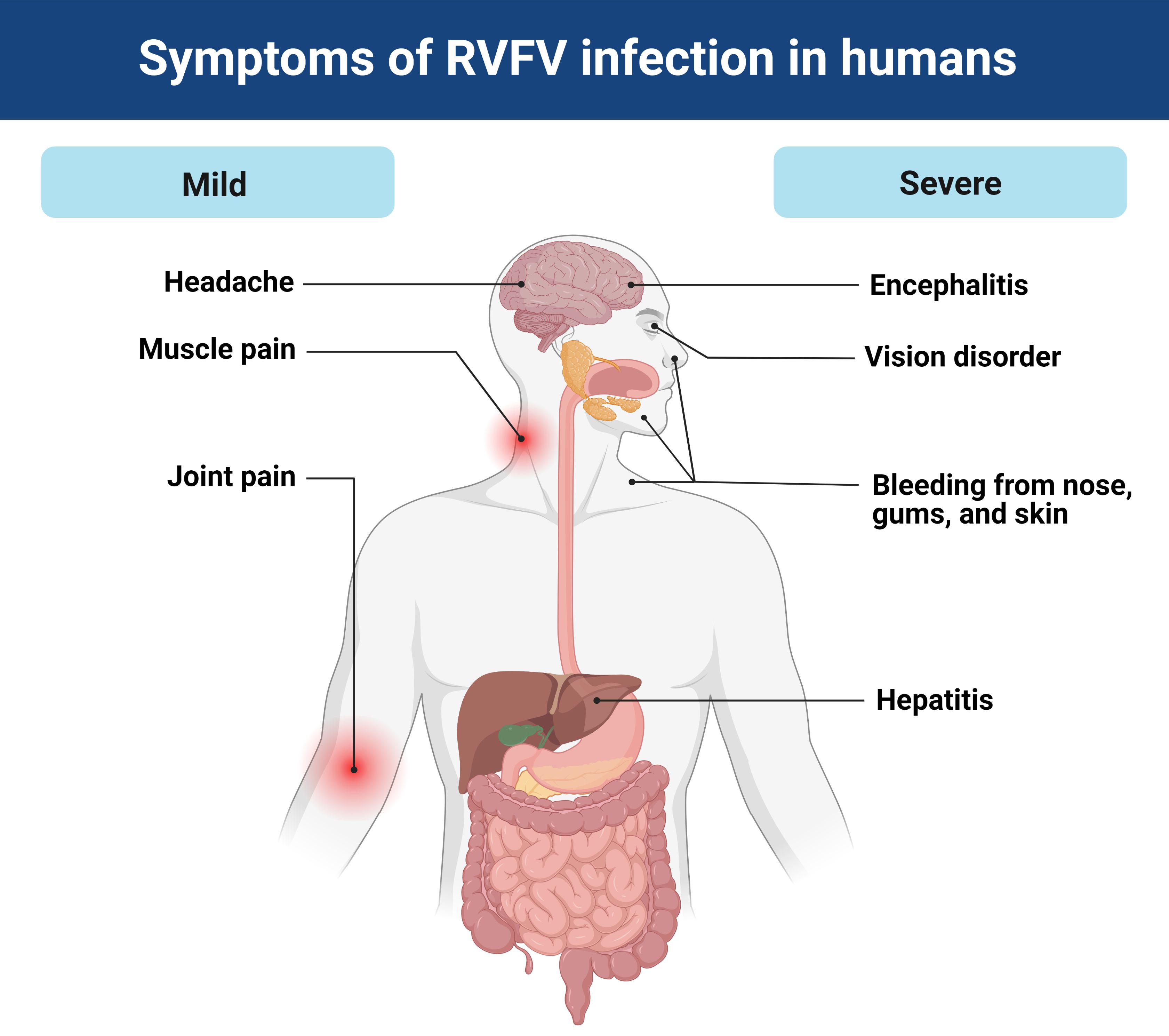
Credit: Abdullah A. Alomar, UF/IFAS
Diagnosis
The diagnosis of RVFV is often challenging because of the broad overlap of symptoms with other viral fevers and diseases. Definitive diagnosis of RVFV involves laboratory testing of blood or tissue samples using one of the following tests: RVFV isolation by cell culture, anti-RVFV IgG and IgM antibody enzyme-linked immunosorbent assay, or RVFV nucleic acid detection by reverse transcriptase polymerase chain reaction assay. Diagnostic testing of RVFV requires a high-containment laboratory at a minimum biosafety level 3-enhanced (e.g., BSL-3 Ag) or BSL-4 due to the aerosolization potential of RVFV and its high transmissibility simply from direct contact to the skin. The necessity for a BSL-3 laboratory poses a major challenge in RVFV diagnostic capacity, given the limited number of these laboratories in endemic regions (Petrova et al. 2020). However, novel rapid test technology with wicking assays opens up the capability to test for RVFV in field-collected mosquito pools (Wanja et al. 2011).
Vaccination
The use of vaccines provides partial or even complete protection against viral infection in animals. Although there are no licensed RVFV vaccines available for human use, several vaccines, such as the live-attenuated Smithburn vaccine, a formalin-inactivated vaccine, and a freeze-dried live attenuated Clone 13 vaccine, are commercially available for veterinary use (Alhaj 2016; Faburay et al. 2017). Vaccines were used successfully to avert a likely major epizootic in 2015/2016 following warnings published in the Rift Valley Fever Monitor (Anyamba et al. 2019). Unfortunately, some RVFV vaccines containing live attenuated virus may also be implicated in outbreaks or contribute to viral reassortment that can complicate the threat of this virus (Campbell et al. 2022; Ikegami 2021).
Prevention and Control
There are several preventive measures that may reduce the burden of RVFV or the risk of RVFV outbreaks, including the implementation of animal immunization before an outbreak occurs to prevent epizootic events (Anyamba et al. 2019). In addition, restriction of livestock movement from infected to uninfected areas (Daubney and Hudson 1931), and establishment of an active animal-health monitoring system (e.g., Lancelot 2009) to provide early warning about new cases can prevent widespread transmission. In humans, due to the absence of a licensed RVFV vaccine, it is recommended that people visiting or living in affected areas avoid contact with body fluids, tissues, or blood of infected animals, including local crafts made from hides or other ungulate tissues. All animal products (e.g., milk and meat) should be thoroughly cooked prior to drinking or eating.
Implementation of integrated mosquito management (IMM) is essential to control mosquito vectors and prevent the spread of mosquito-borne viruses. In countries where RVFV is endemic, this poses a challenge due to the ecology of the RVFV system and the bank of RVFV-infected eggs in flood-prone areas. Once flooding occurs, the application of larvicides can be effective in reducing mosquito density but can be logistically challenging to implement on a large scale (WHO 2018; Anyamba et al. 2010). In non-endemic countries, IMM practices are well suited to preventing RVFV emergence. Prevention can be achieved through surveillance, removal of mosquito habitats (e.g., old tires, plastic covers, buckets, and any other containers that may hold water), control of larval and adult stages through abatement activities (particularly where livestock are abundant), insecticide resistance testing, community involvement, and public education about signs and symptoms of RVF in livestock and humans. Measures to prevent mosquito bites include the application of US Environmental Protection Agency (USEPA)-approved repellents to exposed skin, covering skin by wearing long-sleeved shirts and pants, treatment of clothing with permethrin or other USEPA-approved residuals, and use of bed nets and screens on windows and doors.
Acknowledgments
Map was created by Image Basemap source: Esri, DigitalGlobe, GeoEye, Earthstar Geographics, CNES/Airbus DS, USDA, USGS, AeroGriD, and the GIS User Community. Figures were created by BioRender.com.
References
Abdo‐Salem, S., G. Gerbier, P. Bonnet, M. Al‐Qadasi, A. Tran, E. Thiry, G. Al‐Eryni, and F. Roger. 2006. “Descriptive and Spatial Epidemiology of Rift Valley Fever Outbreak in Yemen 2000–2001.” Annals of the New York Academy of Sciences 1081:240–242. https://doi.org/10.1196/annals.1373.028
Abudurexiti, A., S. Adkins, D. Alioto, S. V. Alkhovsky, T. Avšič-Županc, M. J. Ballinger, D. A. Bente, et al. 2019. “Taxonomy of the Order Bunyavirales: Update 2019.” Archives of Virology 164:1949–1965. https://doi.org/10.1007/s00705-019-04253-6
Ahmed Kamal, S. 2011. “Observations on Rift Valley Fever Virus and Vaccines in Egypt.” Virology Journal 8:1–9. https://doi.org/10.1186/1743-422X-8-532
Alhaj, M., 2016. “Safety and Efficacy Profile of Commercial Veterinary Vaccines Against Rift Valley Fever: A Review Study.” Journal of Immunology Research 1:1–6. https://doi.org/10.1155/2016/7346294
Anyamba, A., J. P. Chretien, S. C. Britch, R. P. Soebiyanto, J. L. Small, R. Jepsen, B. M. Forshey, et al. 2019. “Global Disease Outbreaks Associated with the 2015–2016 El Niño Event.” Scientific Reports 9:1–14. https://doi.org/10.1038/s41598-018-38034-z
Anyamba, A., K. J. Linthicum, J. Small, S. C. Britch, E. Pak, S. de La Rocque, P. Formenty, et al. 2010. “Prediction, Assessment of the Rift Valley Fever Activity in East and Southern Africa 2006–2008 and Possible Vector Control Strategies.” The American Journal of Tropical Medicine and Hygiene 83:43. https://doi.org/10.4269/ajtmh.2010.09-0289
Baba, M., D. K. Masiga, R. Sang, and J. Villinger. 2016. “Has Rift Valley Fever Virus Evolved with Increasing Severity in Human Populations in East Africa?” Emerging Microbes & Infections 5:1–10. https://doi.org/10.1038/emi.2016.57
Bales, J. M., D. S. Powell, L. M. Bethel, D. S. Reed, and A. L. Hartman. 2012. “Choice Of Inbred Rat Strain Impacts Lethality and Disease Course After Respiratory Infection With Rift Valley Fever Virus.” Frontiers in Cellular and Infection Microbiology 2:105. https://doi.org/10.3389/fcimb.2012.00105
Britch, S. C., Y. S. Binepal, M. G. Ruder, H. M. Kariithi, K. J. Linthicum, A. Anyamba, J. L. Small, et al. 2013. “Rift Valley Fever Risk Map Model and Seroprevalence in Selected Wild Ungulates and Camels from Kenya.” PLoS one 8:e66626. https://doi.org/10.1371/journal.pone.0066626
Campbell, C. L., T. K. Snell, S. Bennett, J. H. Wyckoff III, D. Heaslip, J. Flatt, E. K. Harris, et al. 2022. “Safety Study of Rift Valley Fever Human Vaccine Candidate (DDVax) in Mosquitoes.” Transboundary and Emerging Diseases 69:2621–2633. https://doi.org/10.1111/tbed.14415
Centers for Disease Control and Prevention 2020a. “Rift Valley Fever.” (https://www.cdc.gov/vhf/rvf/outbreaks/summaries.html) (access data on November 30, 2022).
Centers for Disease Control and Prevention 2020b. “Rift Valley Fever: Signs and Symptoms.” (https://www.cdc.gov/vhf/rvf/symptoms/index.html) (access data on November 30, 2022).
Chevalier, V., M. Pépin, L. Plee, and R. J. E. S. Lancelot. 2010. Rift Valley Fever—A Threat for Europe?” Eurosurveillance 15:19506. https://doi.org/10.2807/ese.15.10.19506-en
Daubney, R., and J. R. Hudson. 1931. “Enzootic Hepatitis or Rift Valley Fever. An Un-Described Virus Disease of Sheep, Cattle and Man from East Africa.” Journal of Pathology and Bacteriology 34:545–79. https://doi.org/10.1002/path.1700340418
Davies, F. G.. 2010. “The Historical and Recent Impact of Rift Valley Fever in Africa.” The American Journal of Tropical Medicine and Hygiene 83:73. https://doi.org/10.4269/ajtmh.2010.83s2a02
Davies, F. G., K. J. Linthicum, and A. D. James. 1985. “Rainfall and Epizootic Rift Valley Fever.” Bulletin of the World Health Organization 63:941.
El-Akkad, A. M. 1978. “Rift Valley Fever Outbreak in Egypt.” October–December 1977. The Journal of the Egyptian Public Health Association 53:123–128.
Faburay, B., A. D. LaBeaud, D. S. McVey, W. C. Wilson, and J. A. Richt. 2017. “Current Status of Rift Valley Fever Vaccine Development.” Vaccines 5:29. https://doi.org/10.3390/vaccines5030029
Gerdes, G. H. 2004. “Rift Valley Fever. Revue Scientifique et Technique. » International Office of Epizootics 23:613–623. https://doi.org/10.20506/rst.23.2.1500
Gibson, S., K. J. Linthicum, M. J. Turell, and A. Anyamba. 2022. “Rift Valley Fever Virus: Movement of Infected Humans Threatens Global Public Health and Agriculture.” CABI Reviews. https://doi.org/10.1079/cabireviews202217029
Golnar, A. J., M. J. Turell, A. D. LaBeaud, R. C. Kading, and G. I. Hamer. 2014. “Predicting the Mosquito Species and Vertebrate Species Involved in the Theoretical Transmission of Rift Valley Fever Virus in the United States.” PLoS Neglected Tropical Diseases 8:e3163. https://doi.org/10.1371/journal.pntd.0003163
Ikegami, T. 2012. “Molecular Biology and Genetic Diversity of Rift Valley Fever Virus.” Antiviral Research 95:293-310. https://doi.org/10.1016/j.antiviral.2012.06.001
Ikegami, T. 2021. “Development of a Simian RNA Polymerase I Promoter-Driven Reverse Genetics System for the Rescue of Recombinant Rift Valley Fever Virus from Vero Cells.” Journal of Virology 95:e0200420. https://doi.org/10.1128/JVI.02004-20
Ikegami, T., and S. Makino. 2011. “The Pathogenesis of Rift Valley Fever.” Viruses 3:493–519. https://doi.org/10.3390/v3050493
Jupp, P. G., and A. J. Cornel. 1988. “Vector Competence Tests with Rift Valley Fever Virus and Five South African Species of Mosquito.” Journal of the American Mosquito Control Association 4:4–8.
Kwaśnik, M., W. Rożek, and J. Rola. 2021. “Rift Valley Fever—A Growing Threat to Humans and Animals.” Journal of Veterinary Research 65:7–14. https://doi.org/10.2478/jvetres-2021-0009
Linthicum, K. J., A. Anyamba, S. C. Britch, J. P. Chretien, K. E. Bennett, R. T. Mayer, E. T. Schmidtmann, et al. 2007. “A Rift Valley Fever Risk Surveillance System for Africa Using Remotely Sensed Data: Potential for Use on Other Continents.” Veterinaria Italiana 43:663–74.
Linthicum, K. J., A. Anyamba, C. J. Tucker, P. W. Kelley, M. F. Myers, and C. J. Peters. 1999. “Climate and Satellite Indicators to Forecast Rift Valley Fever Epidemics in Kenya.” Science 285:397–400. https://doi.org/10.1126/science.285.5426.397
Linthicum, K. J., S. C. Britch, and A. Anyamba. 2016. “Rift Valley Fever: An Emerging Mosquito-Borne Disease.” Annual Review of Entomology 6:395–415. https://doi.org/10.1146/annurev-ento-010715-023819
Linthicum, K. J., F. G. Davies, C. L. Bailey, and A. Kairo. 1983. “Mosquito Species Succession in a Dambo in an East African Forest.” Mosquito News 43:464–470.
Linthicum, K. J., F. G. Davies, C. L. Bailey, and A. Kairo. 1984. “Mosquito Species Encountered in a Flooded Grassland Dambo in Kenya.” Mosquito News 44:228–232.
Linthicum, K. J., F. G. Davies, A. Kairo, and C. L. Bailey. 1985b. “Rift Valley Fever Virus (family Bunyaviridae, genus Phlebovirus). Isolations from Diptera Collected during an Inter-Epizootic Period in Kenya.” Epidemiology & Infection 95:197–209. https://doi.org/10.1017/S0022172400062434
Linthicum, K. J., H. F. Kaburia, F. G. Davies, and K. J. Lindqvist. 1985a. A Blood Meal Analysis of Engorged Mosquitoes Found in Rift Valley Fever Epizootics Areas in Kenya.” Journal of the American Mosquito Control Association 1:93–5.
Lancelot, R. 2009. “Sentinel Animals in Tropical Environments. Toward an Integrated Surveillance System.” Épidémiologie et Santé Animale 56:27–34.
Logan, T. M., K. J. Linthicum, F. G. Davies, Y. S. Binepal, and C. R. Roberts. 1991. “Isolation of Rift Valley Fever Virus from Mosquitoes (Diptera: Culicidae) Collected during an Outbreak in Domestic Animals in Kenya.” Journal of Medical Entomology 28:293–295. https://doi.org/10.1093/jmedent/28.2.293
Lumley, S., D. L. Horton, L. L. Hernandez-Triana, N. Johnson, A. R. Fooks and R. Hewson. 2017. “Rift Valley Fever Virus: Strategies for Maintenance, Survival and Vertical Transmission in Mosquitoes.” Journal of General Virology 98:875–887. https://doi.org/10.1099/jgv.0.000765
Lutomiah, J., D. Omondi, D. Masiga, C. Mutai, P. O. Mireji, J. Ongus,, K. J. Linthicum, and R. Sang. 2014. “Blood Meal Analysis and Virus Detection in Blood-Fed Mosquitoes Collected during the 2006–2007 Rift Valley Fever Outbreak in Kenya.” Vector-Borne and Zoonotic Diseases 14:656–664. https://doi.org/10.1089/vbz.2013.1564
Madani, T. A., Y. Y. Al-Mazrou, M. H. Al-Jeffri, A. A. Mishkhas, A. M. Al-Rabeah, A. M. Turkistani, M. O. Al-Sayed, et al. 2003. “Rift Valley Fever Epidemic in Saudi Arabia: Epidemiological, Clinical, and Laboratory Characteristics.” Clinical Infectious Diseases 37:1084-1092. https://doi.org/10.1086/378747
Mariner, J. 2018. “Rift Valley Fever Surveillance.” FAO Animal Production and Health Manual No. 21. Food and Agriculture Organization of the United Nations (FAO), Rome, pp. 5, 51.
Meegan, J. M. 1979. “The Rift Valley Fever Epizootic in Egypt 1977–1978 1. Description of the Epizootic and Virological Studies.” Transactions of the Royal Society of Tropical Medicine and Hygiene 73:618–623. https://doi.org/10.1016/0035-9203(79)90004-X
Ndiaye, E. H., G. Fall, A. Gaye, N. S. Bob, C. Talla, C. T. Diagne, D. Diallo, et al. 2016. “Vector Competence of Aedes vexans (Meigen), Culex poicilipes (Theobald) and Cx. quinquefasciatus Say from Senegal for West and East African Lineages of Rift Valley Fever Virus.” Parasites & Vectors 9:1–9. https://doi.org/10.1186/s13071-016-1383-y
Petrova, V., P. Kristiansen, G. Norheim, and S. A. Yimer. 2020. “Rift Valley Fever: Diagnostic Challenges and Investment Needs for Vaccine Development.” BMJ Global Health 5: e002694. https://doi.org/10.1136/bmjgh-2020-002694
Rolin, A. I., L. Berrang-Ford, and M. A. Kulkarni. 2013. “The Risk of Rift Valley Fever Virus Introduction and Establishment in the United States and European Union.” Emerging Microbes & Infections 2:1–8. https://doi.org/10.1038/emi.2013.81
Saluzzo, J. F., J. P. Digoutte, C. Chartier, D. T. Martinez, and R. Bada. 1987. Focus of Rift Valley Fever Virus Transmission in Southern Mauritania.” The Lancet 329:504. https://doi.org/10.1016/S0140-6736(87)92110-6
Shoemaker, T. R., L. Nyakarahuka, S. Balinandi, J. Ojwang, A. Tumusiime, S. Mulei, J. Kyondo, et al. 2019. “First Laboratory-Confirmed Outbreak of Human and Animal Rift Valley Fever Virus in Uganda in 48 Years.” American Journal of Tropical Medicine and Hygiene 100:659–671. https://doi.org/10.4269/ajtmh.18-0732
Sidwell, R. W., and D. F. Smee. 2003. “Viruses of the Bunya-and Togaviridae Families: Potential as Bioterrorism Agents and Means of Control.” Antiviral Research 57:101–111. https://doi.org/10.1016/S0166-3542(02)00203-6
Smithburn, K. C., A. F. Mahaffy, A. J. Haddow, S. F. Kitchen, and J. F. Smith. 1949. “Rift Valley Fever: Accidental Infections among Laboratory Workers.” The Journal of Immunology 62: 213–227. https://doi.org/10.4049/jimmunol.62.2.213
Turell, M. J., K. J. Linthicum, L. A. Patrican, F. G. Davies, A. Kairo, and C. L. Bailey. 2008. “Vector Competence of Selected African Mosquito (Diptera: Culicidae) Species for Rift Valley Fever Virus.” Journal of Medical Entomology 45102–8. https://doi.org/10.1093/jmedent/45.1.102
Turell, M. J., S. M. Presley, A. M. Gad, S. E. Cope, D. J. Dohm, J. C. Morrill, and R. R. Arthur. 1996. “Vector Competence of Egyptian Mosquitoes for Rift Valley Fever Virus.” The American Journal of Tropical Medicine and Hygiene 54:136–9. https://doi.org/10.4269/ajtmh.1996.54.136
Wanja, E., Z. Parker, T. Rowland, M. J. Turell, J. W. Clark, K. Davé, S. Davé and R. Sang. 2011. “Field Evaluation of a Wicking Assay for the Rapid Detection of Rift Valley Fever Viral Antigens in Mosquitoes.” Journal of the American Mosquito Control Association 27:370–375. https://doi.org/10.2987/11-6176.1
Weiss, K. E. 1957. “Wesselsbron Virus Disease.” Bulletin of Epizootic Diseases of Africa 5:459.
Wilkerson, R. C., Y. M. Linton, D. M. Fonseca, T. R. Schultz, D. C. Price, and D. A. Strickman. 2015. “Making Mosquito Taxonomy Useful: A Stable Classification of Tribe Aedini That Balances Utility with Current Knowledge of Evolutionary Relationships.” PloS one 10: e0133602. https://doi.org/10.1371/journal.pone.0133602
World Health Organization. 2018. “Rift Valley Fever Virus.” (https://www.who.int/news-room/fact-sheets/detail/rift-valley-fever) (access data on November 9, 2022).
Youssouf, H., M. Subiros, G. Dennetiere, L. Collet, L. Dommergues, A. Pauvert, P. Rabarison, et al. 2020. “Rift Valley Fever Outbreak, Mayotte, France, 2018–2019.” Emerging Infectious Diseases 26:769. https://doi.org/10.3201/eid2604.191147
Zeller, H. G., D. Fontenille, M. Traoré-Laminzana, Y. Thiongane, and J. P. Digoutte. 1997. “Enzootic Activity of Rift Valley Fever Virus in Senegal.” American Journal of Tropical Medicine and Hygiene 56:265–272. https://doi.org/10.4269/ajtmh.1997.56.265
Zouaghi, K., A. Bouattour, H. Aounallah, R. Surtees, E. Krause, J. Michel, A. Mamlouk, A. Nitsche, and Y. M’ghirbi. 2021. “First Serological Evidence of Crimean-Congo Hemorrhagic Fever Virus and Rift Valley Fever Virus in Ruminants in Tunisia.” Pathogens 10:769. https://doi.org/10.3390/pathogens10060769