Introduction
Water pH is one of the most common water quality measurements made because it influences myriad chemical, physical, and biological processes. The pH of water in an aquatic system is one of the most important water quality parameters because it dramatically influences the bioavailability of some nutrients, metals, and pesticides to plants and animals. It may also influence the bioavailability and fate of several other environmental contaminants.
Objectives
The objectives of this document are to provide managers, students, and technicians with 1) an overview of water pH, 2) an understanding of the importance of pH from ecological and management perspectives, 3) a summary of common methods used to measure pH, and 4) the current state regulations for pH in public waters of Florida. A glossary provided at the end of this document describes terms that are underlined in the text.
Description
The pH of an aqueous solution is often incorrectly defined as the logarithm of the reciprocal of the hydrogen ion (H+) concentration in grams of atoms per liter (moles). However, H+ ions are very reactive, so they are seldom found in the free state. Because of the reactivity of the H+ ions with other ionic constituents in water, it is technically not correct to consider pH as an absolute molar concentration (molar mass, molarity) of hydrogen ions unless the water is pure (APHA et al. 1998). Instead, pH is actually a measure of the H+ "activity" in solution. The activity represents the effective concentration of H+ ions. Thus, pH is best defined as the logarithm of the reciprocal of the H+ activity. The activity is what is measured by pH meters and test kits.
Hydrogen ion activities can range from very low (0.1 x 10-14 moles/L) to very high (0.1 moles/L) in aqueous solutions. The pH naming convention was adopted to facilitate working with such a wide range of numbers. The "p" in "pH" is a standard notation indicating the inverse logarithm of a value (Equations 1 and 2). This naming convention is convenient because it transforms the H+ activities to a decimal scale ranging from 1 to 14 (Table 1). Water having a pH equal to 7 is considered neutral because there are equal activities of H+ and OH- in solution. As the pH is lowered, the H+ ion activity increases, as does the acidity. Conversely, as pH increases, OH- ion activity increases and H+ activity decreases, yielding a basic solution. To transform the pH value to hydrogen ion activity, simply raise 10 to the negative power of the pH (Equation 3).

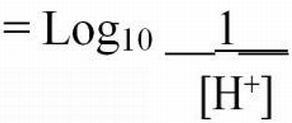
and

Environmental/Management Significance
The pH of soil and water within the environment can significantly influence many different chemical bioavailability and fate processes. The pH of most natural waters falls into the 6 to 9 range due to bicarbonate buffering (see Water Quality Notes: Alkalinity and Hardness for more information on the bicarbonate buffering system https://edis.ifas.ufl.edu/ss540). There are no definite ranges for acceptable pH where fisheries will not be protected or harmed (EIFAC 1969 as reported in Burton Jr. and Pitt 2002). Instead, a pH from 6.5 to 9.0 is tolerated by most fish, although the toxicity of other poisonous constituents may be affected by pH changes within this range (Burton Jr. and Pitt 2002). A pH from 6.0 to 6.5 is not likely to be harmful to fish unless the CO2 concentration is greater than 100 mg/L (ppm) (Burton Jr. and Pitt 2002). Some species are more tolerant of specific pH ranges than others, so these generalizations will not necessarily be representative for all species. A description of some pH effects on pollutants and other environmentally relevant chemicals follows.
Metal Speciation and Bioavailability
The speciation (chemical form), bioavailability, and fate of many metallic pesticides and contaminants in the environment can be significantly affected by pH. In general, free metallic ions (including Cu2+, Mn2+, Fe2+, Co2+, Ni2+, Zn2+, Cd2+, and Pb2+) dominate in acidic aqueous solutions. For example, the free divalent copper ion (Cu2+) is the primary bioactive (toxic) form. In a natural water (buffered by the carbonate system, pH 7, alkalinity = 100 mg/L as CaCO3) only 14% of the total Cu would be in the Cu2+ form. However, as pH increases to 7.5 and 8, only 6% and 2% of the total Cu would be in the bioactive Cu2+ form (Snoeyink and Jenkins 1980). The Cu2+ concentration becomes dominant as the pH approaches 3.
Given the significant impact on bioavailability, special attention should be paid to pH when mixing metallic pesticides with dilution/carrier water since much of the active ingredient can be inactivated (converted to nonbioactive forms) if the pH is not optimal. Consult the product label for recommendations and requirements.
Dissociation of weak acids or bases (NH4+/NH3•nH2O, HCN/CN-, and H2S/S-)
Water pH can have a profound effect on the dissociation of weak acid and base pollutants that vary in toxicity depending on the dominant form. Ammoniacal-N is a nutrient that can be found in natural waters receiving discharges from some agricultural and residential areas, municipal waste treatment plants, and some industries. It is also naturally produced during the decomposition of dead plants and animals. This nutrient can occur in two different chemical forms, ionized (NH4+) and un-ionized (NH3•nH2O), that are in equilibrium with one another (Equation 4).

The relative concentration of each species is a function of pH, temperature, and ionic strength (concentration of dissolved ions). As pH decreases, the concentration of the ionized species (NH4+) increases, while that of the un-ionized species (NH3•nH2O) decreases (Table 2). Conversely, as pH increases, more of the total ammonia will be present in the un-ionized form. The un-ionized form is acutely toxic to many fish and other aquatic animals at low concentrations, whereas the ionized form is not toxic or is appreciably less toxic, depending on the animal species. To put these values into ecological perspective, Russo and Thurston (1991) summarized 96-hour LC50 values for NH3•nH2O, which ranged from 0.08 to 3.8 mg/L for a variety of different fish species. The European Fisheries Advisory Commission (1969) reported that ammonia was 10 times more toxic at pH 8.0 than at pH 7.0 (Burton Jr. and Pitt 2002).
Unlike ammonia, the toxicity of hydrogen cyanide (HCN) and hydrogen sulfide (H2S) increases as pH decreases due to the dominance of the un-ionized species at lower pH values (Equations 5 and 6). As pH increases, both molecules dissociate to form ionized species. As with ammonia, the un-ionized species is able to cross membranes to cause toxicity.


Degradation, Fate, and Bioavailability of Organic Pesticides
Water and soil pH can dramatically impact the fate of some organic pesticides in the environment. Some pesticides degrade more quickly within certain pH ranges and are more stable within others. Several pesticides belonging to the carbamate and organophosphorus chemical families degrade more quickly by hydrolysis at alkaline pH relative to neutral or acidic conditions. For example, fipronil (phenylpyrazole chemical family) has a reported half-life of >100 days at pH 5.5 and 7.0, 32 days at pH 9.0, 4.7 days at pH 10.0, 0.45 days at pH 11.0, and 0.1 days at pH 12.0 (Gunasekara et al. 2007; Bobe et al. 1998).
In addition to influencing pesticide degradation, pH can also significantly influence the solubility and bioavailability of some pesticides. These pesticides have ionization constants, which means that pH can affect their existence as charged or noncharged species. The charge exhibited can significantly affect the water solubility, bioavailability, and exposure route for some pesticides and organisms within aquatic environments. For example, the herbicide bensulfuron-methyl has a water solubility of 120 mg/L at pH 12.0 but only 3.0 mg/L at pH 5 (Wauchope et al. 1992; Hay 1990).
Given these potential influences, special attention should be paid to the pH of pesticide makeup-water and receiving-water bodies (for aquatic-use pesticides) for pH-sensitive products, because the active ingredient may become inactivated or less bioavailable before and during applications. Always consult the product label for recommendations and requirements.
Solubility and Bioavailability of Nutrients
The pH of water and the soil solution can have profound impacts on the bioavailability of nutrients (see also metals as micronutrients). In order for nutrients in the soil to be useful for plant growth and maintenance, they must be dissolved within the soil solution so they can enter and move through the plant. Soil pH is especially important for nutrients such as phosphorus and for micronutrients such as copper, iron, zinc, and aluminum. Inorganic phosphorus forms used by plants include the monovalent H2PO4- and the divalent HPO4-2. Phosphorus is usually taken up rapidly by plants as H2PO4- (Schachtman et al. 1998). HPO4-2 is also absorbed by plants but at a slower rate relative to the monovalent form. Within the soil system, pH controls the relative abundance of the two forms. The monovalent form is more abundant at pH < 7, whereas the divalent form is favored by pH > 7. The micronutrients (also metals) iron, copper, zinc, and aluminum are more water soluble and available for absorption by plants when the pH is relatively acidic. Optimal pH for plant growth ranges from 5 to 6.5 for most plants.
Measurement
Several methods are used to measure water pH. The most common methods use paper test strips or glass electrodes attached to a meter. These two methods are briefly described below.
Test-Strip Method
The pH of water samples can be measured using commercially available test strips (Figure 1). This technique is often used in field sampling water-preservation protocols where nothing should be introduced into the sample container other than the sample. In this case, a small amount of the sample is poured onto a single-use test strip. The test strip is impregnated with pH-active pigments that change color as specified pH values are encountered. Test strips can cover wide or narrow ranges of the pH scale. This method is very inexpensive and simple to execute. However, it is not as precise as the glass electrode method. The accuracy of the measurement depends on the quantitative range and resolution of the test strips.
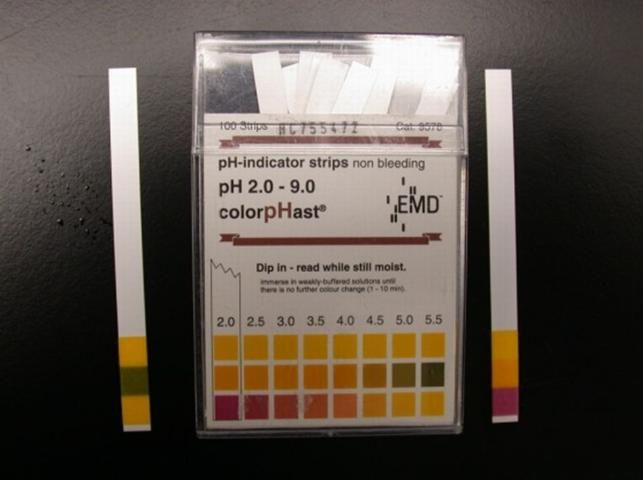
Credit: PCW
Glass Electrode Method
The other common method for measuring pH uses a glass electrode connected to an electronic meter (potentiometer) that measures electromotive force (APHA et al. 1998) (Figure 2A). Electromotive force in this case is the conversion of chemical energy (i.e., H+ activity) into electrical energy that can be measured using the meter. The potentiometer is a device used to measure an unknown voltage by comparison to a standard voltage (Dictionary.com). The glass electrode consists of a bulb (made of a special glass) that contains a fixed concentration of hydrochloric acid (HCl) or a chloride buffer solution in contact with an internal reference electrode (APHA et al. 1998) (Figure 2B). When in contact with the sample, the outer surface of the bulb becomes hydrated and exchanges sodium ions for H+ ions, resulting in a buildup of H+ ions on the surface (APHA et al. 1998). Electrical potential builds up at the glass-solution interface resulting from the H+ ions on the surface and the repulsion of anions by fixed, negatively charged silicate sites in the glass (APHA et al. 1998). The electrical potential produced is a function of the H+ activity in the solution. The meter compares the voltage produced at the glass-surface interface with the standard, known voltage produced by a reference electrode (usually inside the electrode, isolated from the measurement circuit) and then translates the potential into the corresponding pH value using a microprocessor. This method is more sophisticated, requiring the use of more complex devices and calibration standards (Figure 2C). It is also more expensive compared with the test-strip method, but it is more precise.
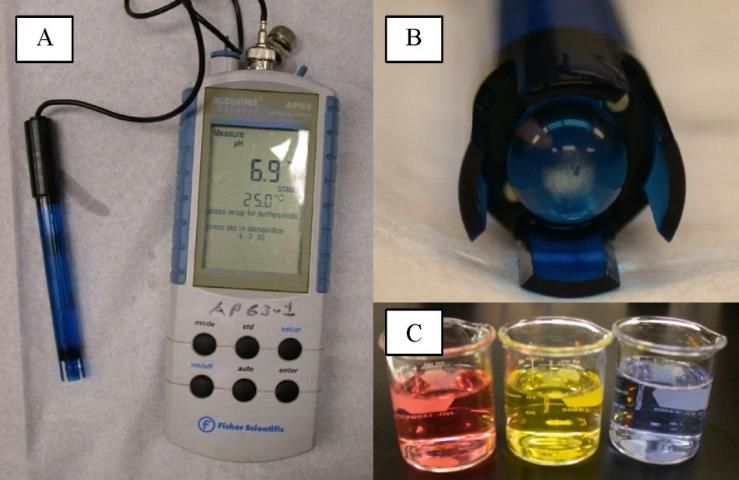
Credit: PCW
Measurement Tips (FDEP 2008, APHA et al. 1998)
- Measurements should be made (in the field) or within 15 minutes of sample collection to minimize errors associated with biological/chemical process-mediated pH changes.
- Calibrate the pH meter as recommended by the manufacturer. For most environmental applications, at least three calibration standards bracketing the sample pH should be used. Standard calibration levels usually include pH 4, 7, and 10, but others may also be used.
- Ensure that readings are stable before calibrating the instrument and before taking readings.
- Ensure that the pH electrode is appropriate for your samples. If pH > 10, standard glass electrodes measure lower than actual pH. In this situation, a "low sodium error" electrode should be used. Likewise, standard glass electrodes yield higher than actual values if pH < 1. In this case, a liquid membrane electrode should be used.
- Longer equilibration times may be needed when measuring the pH of poorly buffered water.
- Thoroughly rinse the electrode between samples and standards using deionized water or calibration buffer solution. Coatings (oil, grease, particulate) will reduce electrode response and increase sample measuring time. Follow manufacturer's recommendations for cleaning.
- If the meter is equipped with temperature compensation, ensure that the feature is activated.
- Always record the temperature of the sample when measuring pH.
Electrode Maintenance Tips
- Always store the electrode as recommended by the manufacturer to extend its useable life span.
- Ensure that buffer levels are correct (if applicable) within the electrode as recommended by the manufacturer.
- Electrodes have a finite, useable life span. Drifting readings and longer equilibration times are often an indicator that the end of the useable life has been reached or that the electrode is fouled.
Florida Surface Water Quality Criteria
The Florida Department of Environmental Protection (FDEP) is charged with protecting water quality within surface water bodies of the state for their respective designated uses. To accomplish this goal, FDEP has defined criteria for pH in state surface water bodies. Table 3 lists the designated uses for each surface water classification group next to FDEP's criteria. Water quality restrictions are greater for numerically lower classes of water (i.e., I > II > III > IV > V). Water flowing from a numerically higher class of water (e.g., class IV) into a more restrictive class (e.g., numerically lower) may be required to meet standards for the lower class.
Related Water Quality Parameters
Alkalinity
Alkalinity is a measure of the acid-neutralizing capacity of a given water sample. It is measured by titrating with a given base to a specific pH endpoint. This property is especially useful for interpretation and control of water and wastewater treatment processes.
Acidity
Acidity is a measure of the base-neutralizing capacity of water. It is measured by titration of the sample with a strong base to a target endpoint pH. This property influences some chemical reaction rates, chemical speciation, biological processes, and corrosivity of the water. It is of primary concern to some manufacturing industries.
References and Suggested Readings
American Public Health Association (APHA), American Water Works Association (AWWA), and the Water Environmental Federation (WEF). 1998. Standard Methods for Examinations of Water and Wastewater, 20th ed. United Book Press, Inc. Baltimore, Maryland.
Bobe, A., P. Meallier, J. F. Cooper, and C. M. Coste. 1998. "Kinetics and mechanisms of abiotic degradation of fipronil (hydrolysis and photolysis)." J. Agric. Food Chem. 46: 2834–2839.
Burton, G. A., Jr., and R. E. Pitt. 2002. Storm Effects Handbook: A Toolbox for Watershed Managers, Scientists, and Engineers. Lewis Publishers, Boca Raton, FL.
"Equilibrium". Dictionary.com. The American Heritage® Science Dictionary. Houghton Mifflin Company. http://dictionary.reference.com/browse/equilibrium (accessed February 11, 2010).
Florida Department of Environmental Protection (FDEP). 2008. DEP-SOP-001/01-FT 1100: Field Measurement of Hydrogen Ion Activity. http://publicfiles.dep.state.fl.us/dear/labs/sas/sopdoc/2008sops/ft1100.pdf.
Gunasekara, A. S., T. Truong, K. S. Goh, F. Spurlock, and R. S. Tjeerdema. 2007. "Environmental fate and toxicology of fipronil." J. Pestic. Sci. 32(3): 189–199.
Hay, J. V. 1990. "Chemistry of sulfonylurea herbicides." Pestic. Sci. 29:247-261.
Kotz, J. C., and K. F. Purcell. 1987. Chemistry and Chemical Reactivity. Saunders College Publishing, Philadelphia, PA.
"Potentiometer". Dictionary.com. The American Heritage® Dictionary of the English Language, Fourth Edition. Houghton Mifflin Company, 2004. http://dictionary.reference.com/browse/potentiometer (accessed: February 02, 2010).
Russo, R. C., and R. V. Thurston. 1991. "Toxicity of ammonia, nitrate, and nitrate to fishes." In Aquaculture and Water Quality. The World Aquaculture Society, Baton Rouge, LA, USA. 59–89.
Schachtman, D. P., R. J. Reid, and S. M. Ayling. 1998. "Phosphorus uptake by plants: from soil to cell." Plant Physiol. 116:447–453.
Snoeyink, V. L., and D. Jenkins. 1980. Water Chemistry. John Wiley & Sons, New York.
Stumm, W., and J. J. Morgan. 1996. Aquatic Chemistry: Chemical Equilibria and Rates in Natural Waters. John Wiley & Sons, Inc., New York.
Wauchope, R. D., T. M. Buttler, A. G. Hornsby, P. W. M. Augustijn-Beckers, and J. P. Burt. 1992. "The SCS/ARS/CES Pesticide Properties Database for Environmental Decision-making." Rev. Environ. Contam. Toxicol. 123: 164.
Glossary
Bioavailability—The degree to which a substance is available for eliciting an effect on an organism. For example, nutrients must be bioavailable to support plant growth, and pesticides must be bioavailable to control target pests.
Mole—The amount of a substance that contains as many elementary particles as there are atoms in 0.0120 kg of carbon-12 isotope. The number of particles in a mole of any substance is 6.022x1023 (Avogadro's number) (Kotz and Purcell 1987).
Molar mass—The mass of 1 mole of atoms of one kind, numerically equivalent to the atomic weight in atomic mass units (amu) (Kotz and Purcell 1987).
Molarity—A measure of solution concentration, given in moles of solute per liter of solution (Kotz and Purcell 1987).
Speciation (chemical)—The particular form in which an element exists in water (Stumm and Morgan 1996).
Equilibrium—A condition in which the forward and reverse reaction rates in a physical or chemical system are equal (Kotz and Purcell 1987).
Hydrolysis—A reaction between an ion and water in which an oxygen-hydrogen bond is broken (Kotz and Purcell 1987).
Ionization constant—A value used to describe the distribution of a chemical that dissociates in solution. For example, HCN + H2O?H3O+ + CN-, the ionization constant (Ka) describes the reaction as
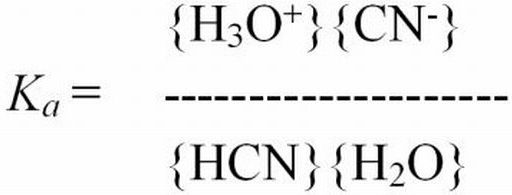
Example of the effect of pH on the amount of total ammonia present in the un-ionized form (NH3•nH2O). Calculations based on a total ammonia (NH4+/NH3•nH2O) concentration of 10 mg/L as N, temperature = 25°C, and salinity = 0. (Reference: http://www.dep.state.fl.us/waste/categories/wc/pages/ProgramTechnicalSupport.htm)
Designated water-use groups and pH criteria for surface waters of the state of Florida. Source: Florida Department of Environmental Protection—https://www.flrules.org/gateway/RuleNo.asp?title=SURFACE%20WATER%20QUALITY%20STANDARDS&ID=62-302.530.