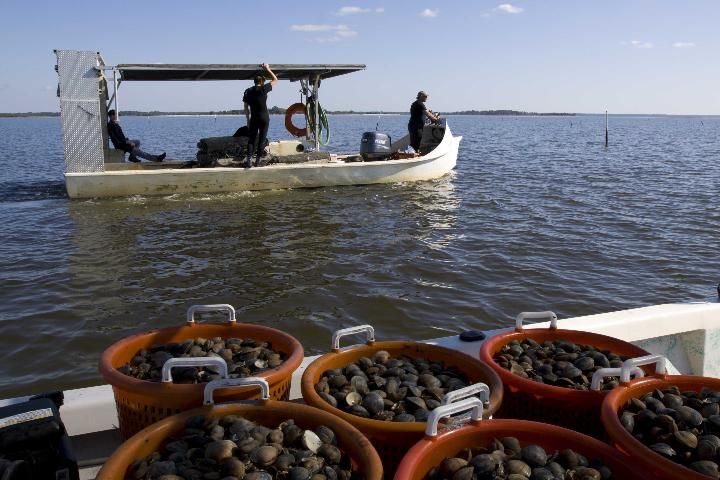
Credit: UF/IFAS photo by Tyler Jones.
What Is Dissolved Oxygen?
Oxygen is a chemical element and a major component (21%) of the air we breathe. This gas is released to the atmosphere by plants during photosynthesis, the process by which light energy and carbon dioxide are converted to food and oxygen (Equation 1). Oxygen is necessary for aerobic respiration in animals, in which energy is released by oxidation of food molecules for use in body maintenance, growth, reproduction, and other activities (Equation 2).
Equation 1: Photosynthesis
carbon dioxide + water + light = food + oxygen + water
Equation 2: Aerobic respiration
food + oxygen = water + carbon dioxide + energy
Oxygen is also present in water, where it is called dissolved oxygen. Most aquatic plants produce oxygen, just as most land plants do; most aquatic animals require oxygen, just as most land animals do.
How Is Dissolved Oxygen Measured?
Dissolved oxygen can be measured by several methods. Unfortunately, measurement of dissolved oxygen requires special, often expensive, equipment. Winkler titration is the most inexpensive method to determine the amount of dissolved oxygen in water, but it is also the least accurate and most labor intensive. Oxygen electrodes and oxygen optodes (different types of oxygen meters) are fast and accurate, but they can be expensive.
Winkler Titration
A water sample is removed from the water body and preserved with chemicals that form a brown precipitate; the amount of precipitate is in direct proportion to the volume of dissolved oxygen present. In the next step, a strong acid is used to convert the precipitate to dissolved iodine. Finally, a titrant solution is slowly added until the brown-black iodine color disappears. The concentration of dissolved oxygen can be calculated from the volume of titrant necessary to make all the color disappear.
Care must be taken to avoid the accidental addition of oxygen to the sample when using this method. Water cannot be simply dipped with a bucket and poured into a bottle because this will aerate, or add oxygen to, the sample, giving a false indication of the amount of dissolved oxygen in the water. Instead, a bottle should be lowered about halfway into the water and allowed to fill slowly. There should be no air bubbles present because air bubbles, too, add oxygen to the water. The stopper of the collection bottle also needs to be carefully placed so that the bottle is completely full of water with no pocket of air or bubbles at the top.
Winkler titration is inexpensive and, despite these necessary precautions, is a relatively easy procedure, especially if you purchase test kits, which are available from aquaculture supply companies. However, these test kits are not as precise or accurate as other methods, and they are more labor intensive.
Oxygen-Sensitive Membrane Electrode
An oxygen-sensitive membrane electrode is a kind of probe that is placed directly into the water. The probe is covered with an oxygen-permeable membrane. The oxygen enters the probe by diffusion across the membrane and is electrolytically reduced (consumed), creating an electrical current. The strength of electrical current is directly proportional to the amount of oxygen entering the probe. Oxygen concentration of the water is read from an attached meter.
Oxygen electrodes are accurate if they are properly calibrated, and they work well under controlled laboratory conditions, but they have several disadvantages for field use. If used continuously in the water ("in situ"), the electrode may become fouled by animals, damaged by debris, or tarnished by hydrogen sulfide, the rotten-egg smelling chemical that comes from the bacterial breakdown of organic matter. Frequent maintenance and cleaning are required for accurate measurements. Oxygen electrodes and meters may be expensive, but economical versions are available for non-continuous use.
Oxygen Optode
An oxygen optode is another oxygen measuring device that is submerged directly in the water. To make an oxygen optode, a chemical film is glued to the tip of a fiber optic cable. The chemical will fluoresce (or light up) to a greater or lesser degree depending on the oxygen concentration in the water. The optode will glow brightest when there is no oxygen present and will dim whenever an oxygen molecule collides with the chemical film. Again, oxygen concentration of the water is read from an attached meter. Oxygen optodes and meters are precise and easy to use, but they are expensive.
Units of Oxygen Measurements
The amount of oxygen dissolved in water may be expressed as a concentration (i.e., the weight or volume of oxygen in a volume of water). For example, dissolved oxygen concentration may be expressed as milligrams of oxygen per liter of water (mg/L) or milliliters of oxygen per liter of water (mL/L). These units can be converted using Equations 3 and 4.
Equation 3: Convert from mg/L to mL/L (i.e., oxygen concentration measured in mg/L)
mL/L = mg/L x 0.69978
Example: 5 mg/L oxygen = mL/L = (5 mg/L) x 0.69978 = 3.5 mL/L
Equation 4: Convert from mL/L to mg/L (i.e., oxygen concentration measured in mL/L)
mg/L = mL/L x 1.4290
Example: 3.5 mL/L oxygen = mg/L = (3.5 mL/L) x 1.4290 = 5.0 mg/L
Dissolved oxygen levels may also be reported as percent saturation (i.e., a relative measure of the amount of oxygen dissolved in water). The percent of dissolved oxygen in water varies with temperature and salinity. If water contains the maximum amount of dissolved oxygen it should theoretically hold, it is said to be "saturated" with oxygen. The amount of oxygen that can be dissolved in water is obtained from solubility tables, which can be found at http://www.aquatext.com/tables/oxygen.htm or http://water.usgs.gov/owq/FieldManual/Chapter6/table6.2_7.pdf. The percent saturation compares the amount of oxygen that is measured in the water to the amount of oxygen the water could hold if it were saturated (Equation 5).
Equation 5: Calculate percent oxygen saturation
Percent saturation = (Measured dissolved oxygen concentration/Maximum oxygen concentration from solubility table) x 100
Example: 4.0 mg/L at 30 ppt and 70°F = % Saturation = [(4.0 mg/L) / (7.5 mg/L)] x 100 = 53%
Saltwater (30 ppt) at 70°F (21°C) that contains 7.5 mg/L of dissolved oxygen is 100% saturated with oxygen, whereas saltwater (30 ppt) at 70°F (21°C) that contains 4.0 mg/L is 53% saturated with oxygen. Water with oxygen saturation between 0 and 30% is called hypoxic, and an oxygen saturation of 0% is called anoxic.
Why Is Dissolved Oxygen Variable?
The maximum amount of oxygen that can be dissolved in water depends on the temperature of the water. Warm water holds less dissolved oxygen than cold water; it is "saturated" with less oxygen. This is illustrated by the bubbles that form on the bottom of a pot of water that is brought to a boil; at warmer temperatures, the water holds less dissolved oxygen, and so the oxygen begins to come out of the water, in the form of bubbles. During the summer, bodies of water will contain less dissolved oxygen (mg/L) than in the winter (Table 1, Figure 1A). However, since the maximum amount of oxygen (100% saturation) that can be dissolved in water is also less, oxygen saturation levels in the summer may not be dramatically different from winter oxygen saturation levels (Figure 1B).
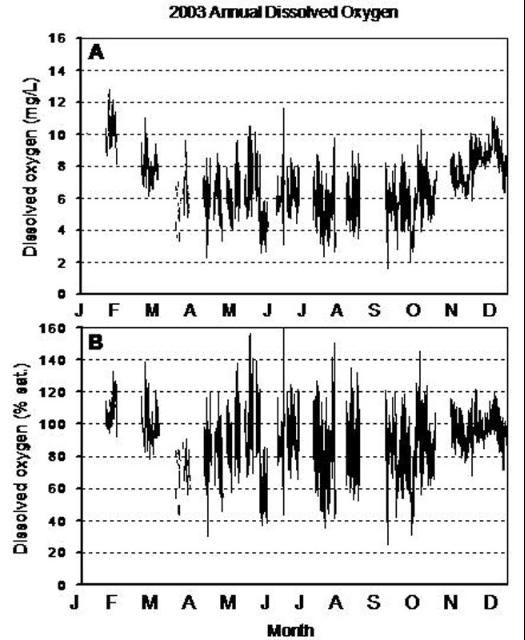
The maximum amount of oxygen that can be dissolved in water also depends on the amount of other substances, such as salts, dissolved in the water. Substances dissolved in the water take up space between the water molecules that could otherwise be occupied by oxygen. Therefore, the water of estuaries holds less oxygen than that of freshwater environments (Table 1).
Both physical and biological processes add dissolved oxygen to water. Physical sources of dissolved oxygen include diffusion of gas from the atmosphere, which is relatively slow, and aeration by wind and wave action. The most important biological source of dissolved oxygen is photosynthesis. During photosynthesis, plants and microscopic algae convert sunlight and nutrients into food, giving off oxygen as a by-product (see Equation 1). Because photosynthesis requires light, it occurs only during daylight hours and can be reduced by cloud cover.
In deep bodies of water, stratification—warmer, less dense water floating on top of colder, denser water at the bottom of a water body—may play a role in distribution of oxygen in the water column. Aerobic respiration and decomposition use up the oxygen in the bottom layer, but the stratification prevents surface water, which is oxygenated by diffusion, aeration, and photosynthesis, from reaching the bottom. As a result, the oxygen concentrations in the top and bottom layers of a stratified body of water may differ considerably (i.e., top water may be fully saturated while the bottom layers may be hypoxic or anoxic). In some situations, stratification can harm the health and productivity of aquatic animals; the section on lease site selection includes further information.
Water sometimes contains more oxygen than it should theoretically hold. This condition is called oxygen supersaturation (>100% saturation). Oxygen supersaturation is primarily caused by rapid solar heating and photosynthesis. Since the solubility of oxygen in water decreases with increasing temperature, supersaturation may occur when surface waters are warmed in the sun more rapidly than the oxygen can escape. In addition, during high levels of oxygen production by plants and algae, dissolved oxygen can far exceed saturation values. Aquatic animals exposed to supersaturated water can develop a condition called gas bubble disease in which bubbles of gas form in the tissues, especially the gills, and may cause death. Gas bubble disease can affect clams in some circumstances; learn about preventative measures in the section on protecting clams from oxygen fluctuations.
Dissolved oxygen is depleted by the biological processes of aerobic respiration and decomposition. Aerobic respiration (see Equation 2) by large numbers of animals, including plankton, removes dissolved oxygen from the water. Decomposition of organic matter by bacteria and fungi also contributes to oxygen depletion. These animals require oxygen to break down organic matter and release nutrients back into the environment. Sources of organic matter include decaying animals, algae, or phytoplankton; urban and agricultural runoff; sewage; and industrial wastes. Decomposition is particularly important in the process of eutrophication. During eutrophication, excess nutrients in the water, usually from runoff or sewage, allow large quantities of algae and plants to grow. When these algae die, they sink to the bottom where bacteria decompose them and use up the oxygen. This leads to hypoxic, or even anoxic, water.
In addition to seasonal variations, diurnal or daily fluctuations in dissolved oxygen are common (Figure 2). During daylight hours, photosynthesis produces oxygen faster than it is used in aerobic respiration, so dissolved oxygen remains high. However, at night, the only source of dissolved oxygen is diffusion, which is rarely as great as respiratory demands, so dissolved oxygen concentrations will decline. Therefore, the lowest oxygen concentrations over a 24-hour period can be expected to occur in the early morning hours, prior to sunrise (Figure 3).
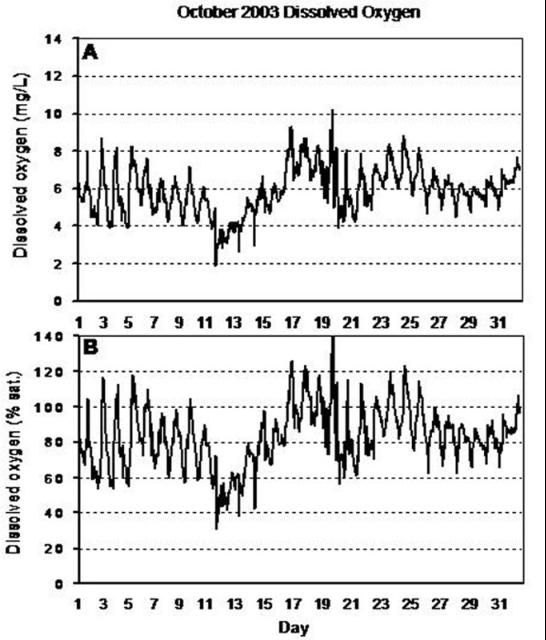
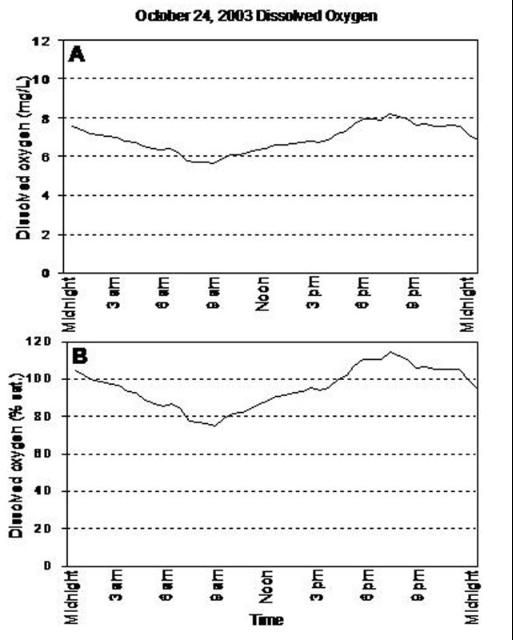
Overview of Hard Clam Production
Hard clam production has three culture stages—production of small seed in a hatchery, growth of larger seed in a land-based nursery and/or field nursery, and growout to marketable size on an open-water lease.
Hatchery—Clam culture begins in the hatchery with the production of seed. In the hatchery, adult clams are induced to spawn by altering the temperature of the water. Fertilized eggs and resulting free-swimming larval stages are reared under controlled conditions in large, cylindrical tanks filled with filtered, sterilized seawater. Larvae are fed cultured phytoplankton (microscopic marine algae) during a 10 to 14-day larval culture phase. After approximately 2 weeks, the larvae begin to settle out of the water column and metamorphose into juvenile clams. Even though a true shell is formed at this time, post-set seed are still microscopic and vulnerable to fluctuating environmental conditions. Thus, they are maintained in downwellers at the hatchery for another 30 to 60 days until they reach about 1 mm in size.
Nursery—The land-based nursery protects small seed until they are ready to be planted out onto the lease for growout. Nursery systems built on land usually consist of weller systems or raceways. Water, pumped from an adjacent saltwater source, provides naturally occurring phytoplankton and oxygen to the clam seed. Depending on water temperatures, 1–2 mm seed, obtained from the hatchery, require from 88 to 12 weeks to reach 5–6 mm in shell length, the minimum size planted in the field.
Growout—Clams are primarily grown on estuarine or coastal submerged lands leased from the State of Florida. Since clams are bottom-dwelling animals, growout systems are designed to place the clam seed on the bottom and provide protection from predators. Most clam growers in the state use a soft bag of polyester mesh material. The bag is staked to the bottom and naturally occurring sediments serve as the bottom substrate. Bag culture usually involves a 2-step process. The first step entails field nursing seed with shell lengths of 5–6 mm (1/4 inch) in a small-mesh bag. After about 3–6 months, the seed reach a growout size of 12–15 mm shell length (1/2 inch) and they are transferred to a bag of larger mesh size. A crop of littleneck clams (25 mm or 1-inch shell width) can be grown in 12–18 months.
How Does Dissolved Oxygen Affect the Physiology of Hard Clams?
Hard clams, like other animals, require oxygen for aerobic respiration. They extract, or "breathe," oxygen from the water with gills, which have large surface areas and very thin epithelia ("skins"). When the clam is filtering or "open," water is moved over the surface of the gills by tiny cilia (small hair-like projections from the gills). Oxygen is transferred from the water to the clam's circulatory system.
If the oxygen concentration of the water becomes too low (hypoxic or anoxic), there will not be a great enough difference in concentration between the water and the clam's circulatory system, so the transfer of oxygen will cease. At this point, the clam will close its valve, or shell, and begin to use anaerobic metabolism, converting fuel reserves to energy without using oxygen. Like many invertebrates, hard clams have outstanding anaerobic capacities. However, anaerobic metabolism produces only a fraction of the energy per unit of fuel that aerobic metabolism does (only about 6%). Therefore, to reduce consumption of fuel reserves and make reserves last longer, hard clams reduce their metabolic rate by as much as 95%. While their shells are closed and metabolic rates are low, however, clams are not feeding, growing, or putting energy into reproduction. This means that persistent low oxygen concentrations can lead to a reduction of biomass and, eventually, death.
What Are Signs of Oxygen Stress?
In the natural environment, it may be difficult to identify oxygen stress in hard clams. Clams can close their valves in response to hypoxic or anoxic conditions and can keep their valves closed for several days. Long-term responses may include gaping of the valves. Signs of adverse environmental conditions in juvenile or adult hard clams may go unnoticed because they are infaunal, which means that they live buried in the sediment. However, stressed clams may rise to the surface of the sediment or fail to bury. These signs are not necessarily specific indications of oxygen stress; they may also be associated with infectious or noninfectious diseases or other adverse environmental conditions such as high temperature and low salinity.
How Does Dissolved Oxygen Affect Hard Clam Production?
Hard clams are very tolerant of low dissolved oxygen. Adult hard clams can maintain aerobic respiration down to dissolved oxygen concentrations of about 4.8 mg/L (64% saturation at 75°F and 20 ppt). At dissolved oxygen levels lower than about 4.8 mg/L, aerobic metabolism declines and anaerobic metabolism becomes increasingly important. Although some activity can be maintained even at dissolved oxygen levels as low as 1.4 mg/L (19% saturation at 75°F and 20 ppt), these levels clearly cause stress to the clams. Under these conditions, growth would be dramatically reduced.
Other environmental conditions affect the ability of clams to survive hypoxic or anoxic conditions, including temperature and salinity. For example, high temperatures (greater than 32°C or 90°F) and low salinity (less than 20 ppt) will intensify the effects of low oxygen concentrations. Low oxygen is especially problematic in the summer months because warmer water not only contains less oxygen, but also stimulates clam's metabolic rates and increases their demand for oxygen. Furthermore, physiological condition (energy stores and spawning stage), age, and size also determine the tolerance of a clam to low oxygen. For example, our laboratory studies indicate that larger clams (1-inch to 2 3/8-inch shell length) can withstand longer periods of low dissolved oxygen than smaller clams (5/8-inch to 1-inch shell length). Likewise, healthier clams are better able to withstand periods of low dissolved oxygen than diseased or stressed clams.
How Can I Manage My Crop in Response to Dissolved Oxygen?
Nurse Clam Seed at Optimal Dissolved Oxygen Concentrations
Low dissolved oxygen is generally not a problem at most land-based nurseries. Water, pumped from an adjacent saltwater source, is aerated as it enters the raceways or downwellers through spray bars, providing adequate oxygen to the clam seed. However, problems may occur when water temperatures are high or when water flow is disrupted. Maintaining a low biomass of seed, especially in the summer months, will prevent the water from becoming hypoxic due to the consumption of oxygen by the animals. In the event of a power outage or pump malfunction, seed can survive several hours without aeration. If the problem is prolonged, then the nursery operator must either provide supplemental aeration with aerators or install a generator. Alternatively, the nursery operator could remove seed from the systems and hold them temporarily (up to 48 hours) in a cooler at temperatures of 65°F-75°F. Seed should be kept drained so they are not sitting in water but should not be allowed to dry out. Seed should also be protected from direct ice exposure.
Gas bubble disease can potentially be a problem in hatcheries and land-based nurseries. Water drawn from the adjacent saltwater source could be supersaturated under some conditions, which might cause gas bubble disease in the clam seed. The simplest way to prevent supersaturation in the hatchery or nursery is to splash or cascade the water as it enters the tank, weller, or raceway system. This will agitate the water and allow the release of excess gas to the atmosphere. Fortunately, clams in the natural environment are not typically susceptible to gas supersaturation.
Consider Dissolved Oxygen Regime in Selecting a Lease Site
Consider salinity and temperature regimes first when you evaluate nursery or growout locations for suitable environmental factors. Dissolved oxygen also plays an important role in the growth and survival of hard clams and should also be considered as you evaluate nursery and growout sites.
In addition to salinity, temperature, and other phenomena already discussed, two physical factors of water bodies, depth and water flow, can either contribute to or offset dissolved oxygen problems and these, too, should be considered in site selection. Sites that are very deep may periodically experience stratification. Stratification occurs when water of high and low salinity, or cold and warm temperature, form layers that act as barriers to mixing. Thermal stratification can become problematic during hot weather, as surface water warms up more rapidly than deep water. The water below the thermocline may become anoxic as organisms use up the oxygen in it. The thermocline acts as a barrier, preventing sufficient mixing between the layers. The water below the thermocline may have too little oxygen to support optimal clam growth, and therefore deep water can possibly be problematic for clam growers during certain times of the year.
Water flow should also be considered when selecting a site. Low dissolved oxygen will be of greater concern in areas that are stagnant or that receive very little water exchange. Water flow and tidal exchange help aerate the water, preventing hypoxia. Most inshore waters, however, should have acceptable dissolved oxygen levels.
Understand the Dissolved Oxygen Regime at Your Site
To manage a clam crop proactively, it is important to understand the dissolved oxygen regime at a given nursery or growout lease site. To better understand and respond to daily, seasonal, and annual variations in dissolved oxygen, growers should take frequent oxygen measurements, as well as record their farming activities and subsequent crop survival.
Taking dissolved oxygen measurements with an electronic meter or test kit over a 24-hour period (diurnal) and during a daily tidal cycle will allow the grower to better understand the fluctuations in oxygen at a site. Growers can expect dissolved oxygen to be lowest in the early morning hours in the summer months. However, taking one measurement will not provide adequate information for a grower to determine if dissolved oxygen levels at the site are a problem.
Historical dissolved oxygen records may also prove useful. Monthly water quality data can be obtained for shellfish harvesting areas in Florida by contacting a Shellfish Environmental Assessment Section (SEAS) field office of the Florida Department of Agriculture and Consumer Services, Division of Aquaculture (see https://www.fdacs.gov/Agriculture-Industry/Aquaculture/Shellfish-Harvesting-Area-Classification). However, it should be noted that these data are recorded during daylight hours. Archived continuous water quality data, collected during 2002-23 at selected aquaculture lease areas in 9 coastal counties can be found at http://shellfish.ifas.ufl.edu/water-quality-monitoring/.
Conduct Growout Activities with Dissolved Oxygen in Mind
In the subtropical climate of Florida, seed clams can be purchased, planted, and transferred throughout the year. However, dissolved oxygen should be considered, especially in the summer when the warm waters of coastal Florida contain less dissolved oxygen. Low oxygen levels may be most problematic on still and overcast days when the addition of dissolved oxygen by physical mixing and photosynthesis are both at a minimum.
Fouling of growout bags and cover nets by encrusting animals, including sea squirts (tunicates) and oyster spat, will reduce the flow of water and dissolved oxygen to the clams. Therefore, growers could minimize the effects of fouling by cleaning the bags of encrusting animals and by removing or replacing cover nets. Growers may also consider using growout bags with a larger mesh size to increase flow.
Clams respire aerobically, consuming oxygen, and therefore the clams themselves may contribute to hypoxic conditions. Larger clams use more oxygen than do small clams. Growers might offset this problem by reducing stocking density and increasing mesh size, especially for larger clams that will remain in growout bags over the summer. Growers might also consider harvesting their largest clams in the spring in anticipation of the potential impacts of low dissolved oxygen in the summer months.
In southwest and east central Florida, macroalgae (drift algae or "rolling moss") can become a problem in the summer months. Dense mats of aquatic vegetation cover growout bags and cover nets and prevent the flow of water and oxygen to the clams, suffocating them. In addition, bacterial decomposition of the algae consumes oxygen and may result in hypoxia. Growers should collect the drift algae and remove it from the lease area. Some growers remove macroalgae with push nets attached to a boat.
Summary
Dissolved oxygen in clam leases is an environmental factor that affects clam survival and growth. Clam growers cannot control dissolved oxygen levels on their leases, so they must consider it when selecting their sites and develop management strategies that adapt to it. The essential first step is dissolved oxygen monitoring; with this information the clam grower can evaluate lease quality and take steps to reduce the impact of dissolved oxygen. To minimize the potential economic impact to the industry, it is prudent to be aware of environmental conditions and be prepared to properly assess any instances of mortality. Assistance from UF/IFAS Extension shellfish and aquatic animal health specialists is available.
State Specialists
Leslie Sturmer — UF/IFAS Extension Shellfish Agent
UF/IFAS Extension, Cedar Key, FL 32625
Phone: (352) 543-5057 — E-mail: LNST@ufl.edu
* * *
Shirley Baker — Professor
UF/IFAS School of Forest, Fisheries, and Geomatics Sciences
7922 NW 71st St, Gainesville, Florida
Phone: (352) 273-3627 — E-mail: sbaker25@ufl.edu
Glossary of Terms Used
Aeration—The process by which air is mixed with or dissolved in water
Aerobic—Requiring oxygen
Aerobic metabolism or aerobic respiration—Cellular reactions requiring oxygen to produce energy from food molecules
Anaerobic energy metabolism—Cellular reactions producing energy from energy stores in the absence of oxygen. Anaerobic metabolism produces far less energy per food molecule than does aerobic metabolism
Anoxia—Complete lack of oxygen
Aquatic—Refers to animals or plants that live in water
Calibration—The process of adjusting the output of a measuring instrument to agree with the value of a known standard
Cilia—Thin hair-like protrusions of cells that beat in coordinated waves to move water and create currents
Circulatory system—An organ system that moves substances to and from cells. Clams have an open circulatory system in which blood in a cavity bathes the organs directly; there are no blood vessels
Concentration—The measure of how much of a given substance there is mixed with another substance
Decomposition—Breaking down of organic matter by bacteria
Diurnal—Daily; cycles that recur every 24 hours are said to be diurnal
Diffusion—A net movement of particles from areas of high concentration to areas of low concentration until equilibrium is reached
Dissolved oxygen—Refers to oxygen in an aqueous medium such as water
Downweller—An open-ended cylinder in which clam seed are suspended on a screen and water flows down over the animals.
Drift algae or "rolling moss"—Macrophytic aquatic plants and seaweed that accumulate in mats as they drift with the currents
Epithelium—A tissue composed of a layer of cells; a "skin." Lines the outside and inside cavities of bodies
Estuary—A coastal body of water with rivers or streams flowing into it and having water that is generally not as salty as sea water
Eutrophication—Refers to an increase in nutrients promoting excessive primary productivity (excessive plant growth and decay) and resulting in a variety of problems such as lack of oxygen in the water
Fluorescence—Light energy emitted in the visible range by a chemical reaction
Fouling or encrusting animals—Animals that attach to hard surfaces
Gas bubble disease—Accumulation of gasses in the tissues of shellfish or fish caused by exposure to supersaturated water
Growout-size clam seed—Refers to clams greater than 10 mm in shell length that are grown on open-water leases in large mesh bags
Hydrogen sulfide—A chemical, smelling of rotten eggs, that results from the breakdown of organic matter by bacteria in the absence of oxygen
Hypoxia—Reduced or inadequate concentration of dissolved oxygen in water
Infaunal—Living in the substrate, usually a soft sediment
Larva—Immature state of an animal that differs markedly in structure from the adult
Metabolism—The complete set of biochemical reactions that takes place in cells, allowing animals to grow, reproduce, and respond to their environment
Metabolic rate—The rate at which food is converted to energy; the amount of energy expended in a given period; or the rate at which oxygen is used in aerobic metabolism
Metamorphosis—The marked and rapid transformation of a larva into an adult form
Molecules—Groups of at least two atoms held together in a particular form by strong chemical bonds
Nutrient—A chemical used by plants and animals. Nutrients required by plants include nitrogen and phosphorus. An oversupply of these plant nutrients can cause excessive plant and algae growth, leading to eutrophication
Organic matter—Material that has come from a recently living animal or plant and can decompose or is the product of decomposition
Oxidation—In aerobic respiration, the addition of oxygen to fuel molecules to produce carbon dioxide and water
Oxygen—One of two major components of air. It is produced during photosynthesis and is necessary for aerobic respiration in animals
Oxygen electrode—A sensor based on the electrochemical consumption of dissolved oxygen
Oxygen optode—A sensor based on optical measurement of dissolved oxygen
Oxygen-permeable membrane—A thin sheet of material that allows oxygen to pass through by diffusion
Photosynthesis—The production of food from sunlight, carbon dioxide, and water. Oxygen is produced
Phytoplankton—Freely floating microscopic aquatic plants (algae) that use phytosynthesis to obtain energy
Plankton—Any drifting organism (plant or animal) in water
Precipitate—A solid that forms from a solution due to a chemical reaction
Primary productivity—Production of organic matter by plants and algae through photosynthesis
Raceway—Shallow tank or tray with horizontal flow of seawater
Saturation—The point at which a solution of a substance can dissolve no more of that substance (100% saturation)
Seed—Typically refers to juvenile clams less than 10 mm in shell length
Signs—Objective evidences of disease
Solution—A homogeneous mixture of one or more substances dissolved in another substance, such as water
Stratification—The formation of layers with warm, less dense water sitting on top of colder, more dense water. Acts as a barrier to water mixing
Supersaturation—Refers to a state in which a solution contains more of a dissolved material than could be dissolved under normal circumstances (> 100% saturation)
Titration—A laboratory method used to determine the concentration of a substance
Titrant—A chemical solution of known concentration and volume that is added to another solution to determine the unknown concentration of a dissolved substance
Thermocline—An area of rapid transition in temperature with depth
Tidal cycle—The cyclic rising and falling of the ocean surface, caused by tidal forces of the moon and sun acting on the oceans, and resulting in changes in depth and oscillating currents
Upweller—An open-ended cylinder in which clam seed are suspended on a screen and water flows up between the animals
Weller system—Consists of open-ended cylinders suspended in a water reservoir or tank. Seawater circulates among the seed clams (either up or down), which are supported by a screen at the bottom of the cylinder
Further Reading
Boyd, C.E. and C.S. Tucker. 1998. Pond Aquaculture Water Quality Management. Kluwer Academic Publishers, Boston. 700 pp.
Goldburg, R. and G.H. Wikfors. 1991. Growth of hard clams in Long Island Sound: sorting out the determining factors. Environ. Manag. 16: 521–529.
Kraeuter, J.N. and M. Castagna. 2001. Biology of the Hard Clam. Elsevier, Amsterdam, Netherlands. 751 pp.
Kraeuter, J.N. and G. Flimlin. 1998. Use of oxygen readings to avoid gas bubble disease in clam hatcheries. New Jersey Sea Grant, Marine Advisory Service Bulletin # 11 (FS906). 4pp.
Malouf, R.E. and V.M. Bricelj. 1989. Comparative biology of clams: environmental tolerances, feeding, and growth. Pages 23-71, In: J.J. Manzi and M. Castagna (Eds.), Clam Mariculture in North America. Elsevier, Amsterdam, Netherlands.
Pratt, D.M. and D.A. Cambell. 1956. Environmental factors affecting growth in Venus mercenaria. Limnol. Oceanogr. 1: 2–17.
Rice, M.A. 1992. The Northern Quahog: The Biology of Mercenaria mercenaria. Rhode Island Sea Grant, Narragansett, RI. 60 pp.
Rice, M.A. and J.A. Pechenik. 1992. A review of the factors influencing the growth of the northern quahog, Mercenaria mercenaria (Linnaeus, 1758). J. Shellfish Res. 11: 279–287.
Roegner, G.C. and R. Mann. 1991. Hard clam, Mercenaria mercenaria. Pages 5.1-5.17, In: S.L. Funderburk, J.A. Mihursky, S.J. Jordan, and D. Riley (Eds.). Habitat requirements for Chesapeake Bay Living Resources. 2nd ed. Chesapeake Research Consortium, Solomons, MD.
Schmidt-Nielsen, K. 1997. Animal Physiology; Adaptation and Environment. 5th ed. Cambridge University Press, New York, NY. 612 pp.
Sturmer, L.N. 2004. Florida Shellfish Aquaculture Extension. http://shellfish.ifas.ufl.edu
Wells, H.W. 1957. Abundance of the hard clam Mercenaria mercenaria in relation to environmental factors. Ecology 38: 123–128.
The maximum amount of oxygen (100% saturation) dissolved in fresh water and sea water at different temperatures, shown as milligrams of oxygen per liter of water (mg/L).